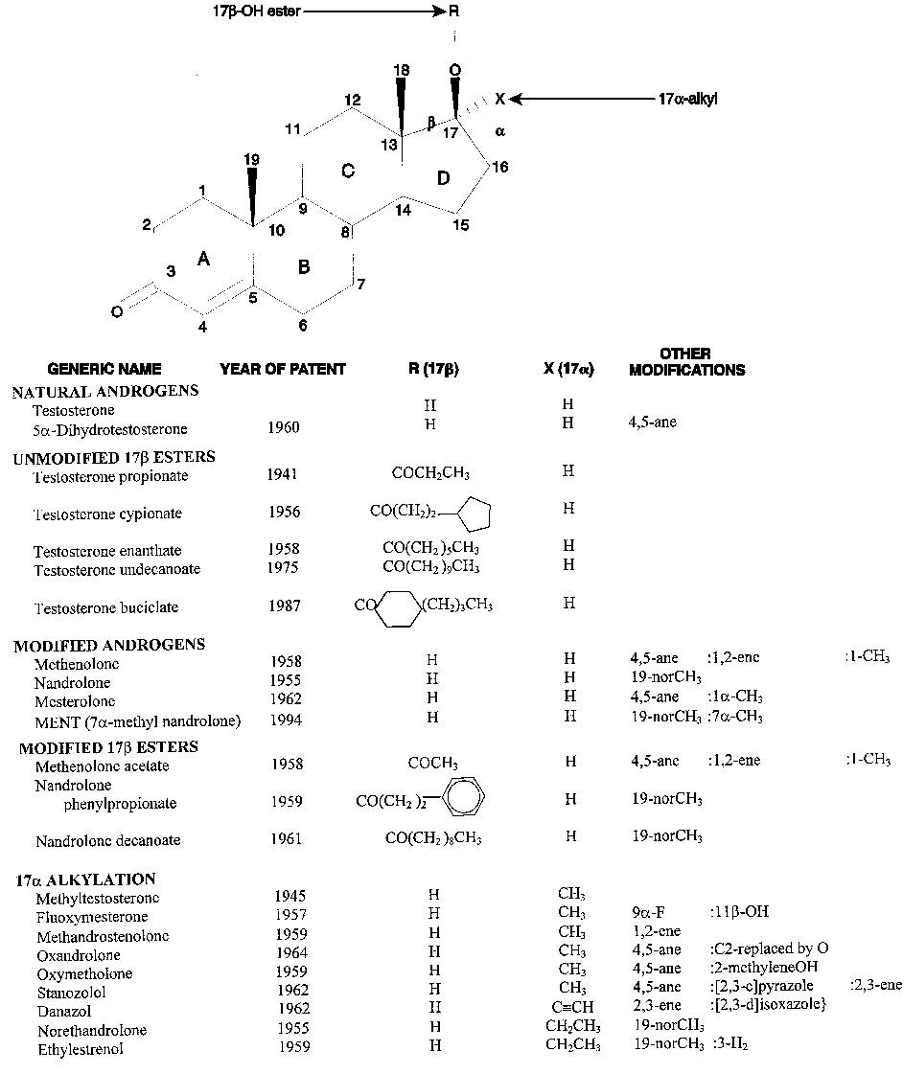
FIGURE 6. Testosterone and its pharmacological derivatives. Listed are the most common synthetic androgens displaying their structural relationship with testosterone.
Steriodal Androgens
Most oral androgens are hepatotoxic 17a-alkylated androgens (methyltestosterone, fluoxymesterone, oxymetholone, oxandrolone, ethylestrenol, stanozolol, danazol, methandrostenolone, norethandrolone) making them unacceptable for long-term androgen replacement therapy. The 1-methyl androgen mesterolone is an orally active DHT analog that undergoes neither amplification by 5a reduction nor aromatization but it is free of hepatotoxicity. Mesterolone is not used for long-term androgen replacement due to the need for multiple daily dosing, its poorly defined pharmacology (691) and suboptimal efficacy at standard dose (570, 577). For historical reasons, the other marketed 1-methyl androgen methenolone is used almost exclusively in anemia due to marrow failure (692, 693) although it has no specific pharmacological advantage over testosterone or other androgens.
Nandrolone (19-nor testosterone) is a widely used injectable androgen in the form of aliphatic fatty acid esters in an oil vehicle mainly for treatment of postmenopusal osteoporosis where it is effective at increasing bone density and reducing fracture rate (694, 695). It is also the most popular androgen abused in sports doping and in body building. Nandrolone is a naturally occuring steroid but is not normally secreted in the human bloodstream although it occurs as an intermediate in the aromatization of testosterone to estradiol by the aromatase enzyme (696). This enzyme complex undertakes two successive hydroxylations on the angular C19 methyl group of testosterone followed by a cleavage of the C10-C19 bond to releases formic acid and aromatize the A ring (697). Nandrolone represents a penultimate step of the molecule undergoing aromatization bound to the enzyme complex with the C19 methyl group excised but a still non-aromatized A ring. Paradoxically, despite being an intermediate in the aromatization reaction, nandrolone is virtually not aromatized after parenteral administration in men (698, 699), presumably because it is a very poor substrate for the human aromatase enzyme (700). It is susceptible to amplification by 5a reductase with its 5a reduced metabolites being moderately activated in androgenic potency (701). The minimal aromatizability of nandrolone makes it suitable for treatment of osteoporosis in women in whom estrogen therapy is contraindicated due to hormone sensitive cancers (breast, uterus) or for older women, although virilization limits its acceptability (702).
Synthetic nandrolone derivatives 7a-methyl 19-nortestosterone (MENT) (703) and 7a, 11b-dimethyl 19-nortestosterone (dimethandrolone) (704) are potent, non-hepatotoxic androgens. MENT is being developed as a depot androgen (705) for androgen replacement (706) and male contraception in an androgen-progestin combination regimen (707) while dimethandrolone has potential for male contraception as a single steroid with dual androgen and progestin activity (708). As nandrolone derivatives, these synthetic androgens are less susceptible to amplification by 5a-reduction (700, 709) whereby their 5a-reduced metabolites have reduced AR binding affinity (710). Disparities in reported susceptibility to aromatization vary from minimal using a recombinant human aromatase assay (700)whereas greater aromatization is reported using purified human or equine placental aromatase (709, 711, 712). The inability of MENT to maintain bone density in androgen deficient men (575) may be due to underdosing rather than an intrinsic feature of this synthetic androgen but illustrates the need for thorough dose titration in different tissues for synthetic androgens that may not possess the full characteristic spectrum of testosterone effects.
Nonsteroidal Androgens
The first nonsteroidal androgen was reported in 1998 (582) and the first placebo-controlled randomized clinical trial in 2013 (713). None are yet approved for clinical use but registration studies are underway for enobosarm (714). Based on structural modifications of the nonsteroidal class of the aryl-propionamide antiandrogens (bicalutamide, flutamide), these compounds offer the possibility of orally active, potent androgens. Subsequently, additional classes of non-steroidal androgen based on structures including quinolines, hydantoins, tetracyclic indoles and oxachrysensones have been reported. Lacking the classical steroid ring structure such androgens are likely to be not subject to androgen activation either by 5a reductase or aromatization but, if taken orally, subject to first-pass hepatic metabolism. Such hepatic metabolism can eliminate in vivo bioactivity of analogs with potent in vitro androgenic effects (715) whereas metabolically resistant analogs can produce potent and disproportionate androgenic effects on the liver in transit. Many of the novel non-steroidal androgens demonstrate potent androgenic effects experimentally on muscle, bone and sexual function while minimizing prostate effects in experimental animals but none have yet undergone full clinical evaluation. These selective effects may be attributable to the tissue-selective distribution of 5a-reductase as a local tissue, pre-receptor androgen amplification mechanism (716) or more complex mechanisms involving ligand-induced receptor conformation changes and/or post-receptor co-regulator interaction mechanisms such as define the tissue selectivity and agonist/antagonist specificity of non-steroidal estrogen partial agonists (717). These features suggest that non-steroidal androgens have potential for development into pharmacologic androgen therapy regimens as tissue-selective mixed or partial androgen agonists (“selective androgen receptor modulators”, SARM) (419, 718). Conversely, they are not ideal for androgen replacement therapy where the full spectrum of testosterone effects including aromatization is idealy required, especially for tissues such as the brain (148, 159) and bone (153) where aromatization is a prominent feature of testosterone action. The clinical efficacy and safety of non-steroidal androgens have yet to be reported and none are yet marketed. Whether the hepatotoxicity of antiandrogens (719, 720) will also be a feature of non-steroidal androgens remains to be determined.
Choice of Preparation
The choice of testosterone product for androgen replacement therapy depends on physician experience and patient preference, involving factors such as convenience, availability, familiarity, cost, and tolerance of frequent injections. Preparations of testosterone or its esters are favored over synthetic androgens for all androgen replacement therapy applications by virtue of their long record of safety and efficacy, ease of dose titration and of monitoring of blood levels as well as the possibility that synthetic androgens lack the full spectrum of testosterone effects through pre-receptor tissue activational mechanisms (5a reduction, aromatization). The hepatotoxicity of synthetic 17a-alkylated androgens (340, 341) makes them unsuitable for long-term androgen replacement therapy.
Cross-over studies indicate that patients prefer testosterone formulations that maintain stable blood levels and smoother clinical effects. This is best achieved by testosterone products that form effective depots for sustained release such as long-acting testosterone implants (6 monthly) (657) and injectable testosterone undecanoate (3 monthly) (721, 722) or shorter-acting daily transdermal gels (71). These are an improvement over the previous standard of intramuscular injections of older testosterone esters (enanthate, mixed esters) in an oil vehicle every 2-3 weeks (655, 657, 658) which produce characteristically wide fluctuations in testosterone levels and corresponding roller-coaster symptomatic effects.
There are few well-established formulation or route-dependent differences between various testosterone products once adequate doses are administered. As with estrogen replacement (723, 724), testosterone effects on SHBG are effectively manifestations of hepatic overdose (725) so that oral ingestion of either 17a-alkylated androgens (726) or oral testosterone undecanoate (657) cause prominent lowering of SHBG levels due to prominent first-pass hepatic effects. By contrast long or short acting sustained-action testosterone depot products cause at most minor transient decreases, mirroring blood testosterone levels, or no effects on blood SHBG (590, 639, 657, 660, 721). The more convenient and well tolerated depot testosterone products which maintain steady-state delivery patterns (71, 590, 657, 721, 722) are supplanting the older, short-term (2-3 week) injectable testosterone esters (enanthate, mixed esters) as the mainstays of androgen replacement therapy.
Side Effects of Androgen Therapy
See also Endotext, Endocrinology of Male Reproduction, Androgens and Cardiovascular Disease in Men
Serious adverse effects from androgen replacement therapy using physiological testosterone doses for appropriate indications are rare. This corresponds to the observation that testosterone is the only hormone without a well defined, spontaneously occurring clinical syndrome of hormone excess in men. However, supraphysiological doses of synthetic androgens in pharmacological androgen therapy or the massive doses of androgen abusers as well as unphysiological use of androgens in chidren or women may produce unwanted androgenic side effects. Oral 17aalkylated androgens also risk a wide range of hepatic adverse effects. Virtually all androgenic side effects are rapidly reversible on cessation of treatment apart from inappropriate virilization in children or women in which voice deepening, terminal body hair, or stunting of final height may be irreversible.
STEROIDAL EFFECTS
Androgen replacement therapy activates physical and mental activity to enhance mood, behavior, and libido, thereby reversing their impairment during androgen deficiency (727). In otherwise healthy men, however, additional testosterone at doses equivalent to testosterone replacement doses (eg for male contraception or “andropause”) mood or behaviour changes are not evident (354, 728-734) or minimal (669). Even among healthy young men having very high androgen doses there are few mood or behaviour changes (488, 735-738) except for a small minority (~5%) of paid clinical trial vounteers who display a hypomanic reaction, reversible on androgen discontinuation (488). However, such adverse behavioural reactions were not observed in larger studies of testosterone administration to unpaid healthy men (666, 669, 739, 740). The higher prevalence of adverse behavioral effects reported among androgen abusers may be related not only to the massive androgen doses but also to high levels of background psychological disturbance (527), drug habituation (557), and anticipation (741) which predispose to behavioral disturbances reported during this form of drug abuse (727, 742).
Excessive or undesirable androgenic effects may be experienced during androgen therapy due to intrinsic androgenic effects in inappropriate settings (e.g., virilization in women or children). In a few untreated hypogonadal men, mainly in newly diagnosed older men, initiation of androgen treatment with standard doses occasionally produces an unfamiliar and even intolerable increase in libido and erection frequency. If this occurs, more gradual acclimatization to full testosterone dose with counseling of men and their partners may occasionally be helpful but usually adequate advice before starting treatment is sufficient.
Seborrhea and acne are commonly associated with high androgen levels in either the steep rise in endogenous testosterone during puberty or among androgen abusers. In contrast to the predominantly facial distribution of adolescent acne, androgen-induced acne with onset well after puberty is characteristically truncal in distribution and provides a useful clinical clue to androgen abuse (743). Acne is unusual during testosterone replacement therapy being mainly restricted to a few susceptible individuals during establishment of treatment with shorter-acting intramuscular testosterone esters, probably related to their generation of transient supraphysiologic testosterone concentrations in the days after injection (570, 655). Acne is rare with depot testosterone products that maintain steady-state physiologic blood testosterone levels. Androgen-induced acne is usually adequately managed with topical measures and/or broad-spectrum antibiotics, if required, with either dose reduction or a switch to steady-state delivery (gel, long-acting injectable) that avoids supraphysiologic peak blood testosterone concentrations. Increased body hair and temporal hair loss or balding may also be seen even with physiologic testosterone replacement in susceptible men.
Modest weight gain (up to 5kg) reflecting anabolic effects on muscle mass is also common. Gynecomastia is a feature of androgen deficiency but may appear during androgen replacement therapy, especially during use of aromatizable androgens such as testosterone that increase circulating estradiol levels at times when androgenic effects are inadequate (e.g., a too low or infrequent dose or unreliable compliance with treatment).
Obstructive sleep apnea causes a mild lowering of blood testosterone concentrations that is rectified by effective continuous positive airway pressure treatment (744). Although testosterone treatment has precipitated obstructive sleep apnea (745) and has potential adverse effects on sleep in older men (746), the prevalence of obstructive sleep apnea precipitated by testosterone treatment remains unclear. The risk is rare in younger androgen deficient men, but is higher among older men with the steeply rising background prevalence of obstructive sleep apnea with age. Hence, screening for obstructive sleep apnea by asking about daytime sleepiness and partner reports of loud and irregular snoring, especially among overweight men with large collar size, is wise for older men starting testosterone treatment but not routinely required for young men with classic androgen deficiency.
THROMBOEMBOLISM
Testosterone treatment of men without pathological hypogonadism were first reported to be associated with an increased risk of venous thromboembolism in a large UK general practice database (747) especially in the first 3-6 months after starting treatment (747-749). These findings were confirmed in another study (750) whereas other studies (751-754) considering only overall or cumulative thromboembolism risk without distinguishing early from later time periods following start of treatment, did not report an increased risk. The mechanism for early coagulopathies might be underlying thrombophilia-hypofibrinolysis due to Factor V Leiden, high lipoprotein (a) or lupus anticoagulant who are at risk of early thromboembolism (748) with recurrence despite adequate anticoagulation (755); however, whether testosterone treatment increases risk remains unclear. Endogenous testosterone is reportedly a risk factor for thromboembolism in a two sample Mendelian randomization study (756) but not confirmed in a 10-year follow-up of 1350 Norwegian men in a population-based study (757).
HEPATOTOXICITY
Hepatotoxicity is a well-recognized but uncommon side effect of 17a-alkylated (340) whereas the occurrence of liver disorders in patients using non-17a alkylated androgens such as testosterone, nandrolone, and 1-methyl androgens (methenolone, mesterolone) are no more than by chance (341). This is consistent with the evidence of direct toxic effects on liver cells of alkylated but not non-alkylated androgens (758). The risk of 17a alkylated androgen-induced hepatotoxicity is unrelated to the indication for use, although association with certain underlying conditions may be related to intensity of diagnostic surveillance (341). It is possible, but unproven, that the risks are dose-dependent although relatively few cases are reported among women using low dose methyl-testosterone (759, 760) while clinical management of children using the alkylated androgen oxandolone often omits liver function tests. However, even if the risks are dose-dependent, the therapeutic margin is narrow. By contrast, the rates of hepatotoxicity among androgen abusers who typically use supraphysiological, often massive, doses remain difficult to quantify due to underreporting of the extent of illicit usage and dosage but abnormal liver function tests are common in androgen abusers when checked incidentally as part of other health evaluation.
Biochemical hepatotoxicity may involve either a cholestatic or hepatitic pattern and usually abates with cessation of steroid ingestion. Elevation of blood transaminases without gamma-glutamyl transferase may be attributable to rhabdomyolysis rather than to hepatotoxicity if confirmed by increased creatinine kinase (761). Major hepatic abnormalities are related to use of 17-alkylated androgens include peliosis hepatis (blood-filled cysts) (762) and hepatic rupture, adenoma, angiosarcoma (763, 764) and carcinoma; however, these risks do not apply to testosterone or other nonalkylated androgens such as nandrolone or 1-methyl androgens. Prolonged use of 17a-alkylated androgens, if unavoidable, requires regular clinical examination together with biochemical monitoring of hepatic function, the latter not required for non-alkylated androgens. If biochemical abnormalities are detected, treatment with 17a-alkylated androgens should cease and safer androgens may be substituted without concern. Where structural lesions are suspected, radionuclide scan, ultrasonography, or abdominal computed tomography scan should precede hepatic biopsy during which severe bleeding may be provoked in peliosis hepatis. Because equally effective and safer alternatives exist, the hepatotoxic 17a-alkylated androgens should not be used for long-term androgen replacement therapy. By contrast, pharmacological androgen therapy often uses 17a alkylated androgens for historical reasons rather than the non-hepatotoxic alternatives. In these situations, the risk-benefit analysis needs to be judged according to the clinical circumstances.
Complications related to testosterone products may be related to dosage, mode of administration or idiosyncratic reactions to constituents. Intramuscular injections of oil vehicle may cause local pain, bleeding, or bruising and, rarely, coughing fits or fainting due to pulmonary oil microembolization (POME) (765) as a minor variant of accidental self-injection oil embolism (766, 767). In a study of over 3000 consecutive injections by experienced nurses, POME occurred at a rate of ~2% (768) but is often unrecognised or under-reported (769) due to the transient symptoms. There was also no bruising or bleeding reported even among men using anticoagulants and/or antiplatelet drugs (upper confidence limit of risk ~1%) (768). Inadvertent subcutaneous administration of the oil vehicle is highly irritating and may cause pain, inflammation, or even dermal necrosis. Allergy to the vegetable oil vehicle (sesame, castor, arachis) used in testosterone ester injections is very rare, and even patients allergic to peanuts may tolerate arachis (peanut) oil. Self-injection by body-builders of large volumes of sesame or other oils may cause exuberant local injection site reactions (770) or even oil embolism (766). Long-term fibrosis at intramuscular injection sites might be expected but has not been reported. Oral testosterone undecanoate may causes gastrointestinal intolerance due to the castor oil/propylene glycol laurate suspension vehicle. Testosterone implants may be associated with extrusion of implants or bleeding, infection, or scarring at implant sites (594). Parenteral injection of testosterone undecanoate (721) or biodegradable microspheres (640) involves a large injection volume that may cause discomfort. Transdermal patches applied to the trunk cause skin irritation in most men, some with quite severe burn-like lesions (609, 771) with a significant minority (~20%) are unable to continue use . Skin irritation may be reduced in prevalence or ameliorated by concurrent use of topical corticosteroid cream at the application site (610) while transdermal testosterone gels (571) or solution (621) are rarely irritating. Topical testosterone gels can cause virilization via transfer of androgens through topical skin-to-skin contact with children (626-631) or sexual partners (623, 624). These problems can be avoided by covering the application site with clothing or washing off excess gel after a short time (772).
Monitoring of Androgen Replacement Therapy
Monitoring of androgen replacement therapy involves, primarily, clinical observations to optimize androgen effects including ensuring the continuation of treatment and surveillance for side effects. Once testosterone dosage is well established, androgen replacement therapy requires only very limited, judicious use of biochemical testing or hormone assays to verify adequacy of dosage when in doubt or following changes of product or dosage. Testosterone and its esters at conventional doses for replacement therapy are sufficiently safe not to require routine biochemical monitoring of liver, kidney or electrolytes.
Clinical monitoring depends on serial observation of improvement in the key presenting features of androgen deficiency. Androgen-deficient men as a group may report subjective improvement in one or more of a variety of symptoms (some only recognized in retrospect) including energy, well-being, psychosocial drive, initiative, and assertiveness as well as sexual activity (especially libido and ejaculation frequency), increased truncal and facial hair growth and muscular strength and endurance. Individual men will become familiar with their own leading androgen deficiency symptom(s), and these appear in predictable sequence and at consistent blood testosterone thresholds towards the end of any treatment cyce (315, 773). Subjective symptoms of genuine androgen deficiency are alleviated quickly, typically within 3 weeks and reach plateau within 2-3 months (774) whereas persistent symptoms after 3 months may represent placebo responses reflecting the non-specificity of androgen deficiency symptoms and the unusually prominent expectations in the community for testosterone treatment. Objective and sensitive measures of androgen action are highly desirable but not available for most androgen-responsive tissues (775). The main biochemical measures available for monitoring of androgenic effects include hemoglobin and trough reproductive hormone (testosterone, LH, FSH) levels. In androgen deficient men, hemoglobin typically increases by ~10% (or up to 20 g/L) with standard testosterone doses (352, 570, 776). Excessive hemoglobin responses (hematocrit ≥0.54, or ≥0.50 with higher risk of cardio- or ceberebrovascular ischemia) occur as a rare (~1%) idiosyncratic reaction which is more frequent at older age (352) explaining the higher prevalence of polycythemia in older testosterone-treated men (777). Testosterone-induced polycythemia is dose-dependent (352, 778) being related to the supraphysiological peak blood testosterone levels observed with shorter-acting testosterone ester injections (570) or trough blood testosterone during treatment with injectable testosterone (778) although it can occur at high enough androgen doses in older men even with transdermal products (779). Such androgen-induced secondary polycythemia is characteristically negative for JAK2 mutations distinguishing it from primary polycythemia rubra vera (780) and usually resolves with reducing testosterone dose and/or switching to more steady-state testosterone delivery systems (implants, injectable testosterone undecanoate or transdermal gel) (781) and only very rarely is venesection and/or anticoagulation required. Circulating testosterone and gonadotropin levels must be considered in relation to time since last testosterone dose. Trough levels (immediately before next scheduled dose) may be helpful in establishing adequacy of depot testosterone regimens. In the presence of normal testosterone, negative feedback on hypothalamic GnRH and pituitary LH secretion (in men with hypergonadotropic hypogonadism), plasma LH levels are elevated in rough proportion to the degree of androgen deficiency. In severe androgen deficiency, virtually castrate LH levels may be present, and, conversely, circulating LH levels provide a sensitive and specific index of tissue testosterone effects (590, 655) especially with more steady-state testosterone delivery by depot-type products. Suppression of LH into the eugonadal range indicates adequate androgen replacement therapy, whereas persistent nonsuppression after the first few months of treatment is an indication of inadequate dose or pattern of testosterone levels. In hypogonadotropic hypogonadism, however, impaired hypothalamic-pituitary function diminishes circulating LH levels regardless of androgen effects, so blood LH levels do not reflect tissue androgenic effects.
Blood testosterone measurements are valuable before treatment for diagnosis and after start of treatment to check adequacy of dosage if in doubt and, during long-term treatment, only to evaluate changes in treatment dosage or product. During depot testosterone treatment which achieves quasi steady-state blood testosterone levels, trough blood testosterone levels taken prior to the next dose may detect patients whose treatment is suboptimal and whose dose and/or treatment interval need modification. Blood testosterone levels are not helpful for monitoring of oral testosterone undecanoate while pharmacological androgen therapy using any synthetic androgens would lower endogenous blood testosterone levels. Serial evaluation of bone density (especially vertebral trabecular bone) by dual photon absorptiometry at 1- to 2-year intervals may be helpful as a time-integrated measure to verify the adequacy of tissue androgen effects (420, 572).
Although chronic androgen deficiency protects against prostate disease (130, 782, 783), prostate size of androgen-deficient men receiving androgen replacement therapy is restored to, but does not exceed, age-appropriate norms (784, 785). Even prolonged (2 years) high doses of exogenous DHT did not significantly increase age-related prostate growth in middle-aged men without known prostate disease (681). Between-subject variability in response to testosterone replacement is partly explained by genetic sensitivity to testosterone, which is inversely related to length of the CAG triplet (polyglutamine) repeat polymorphism in exon 1 of the androgen receptor (235). Furthermore, because neither endogenous blood testosterone nor circulating levels of other androgen predicts subsequent development of prostate cancer (454), maintaining physiologic testosterone concentrations should ensure no higher rates of prostate disease than eugonadal men of similar age (786).
The potential long-term risks for cardiovascular disease of androgen replacement and pharmacologic androgen therapy remain uncertain. Although men have two to three times the prevalence (430) as well as earlier onset and more severe atherosclerotic cardiovascular disease than women, the precise role of blood testosterone and of androgen treatment in this marked gender disparity is still poorly understood (309). Although low blood testosterone concentration is a risk factor for cardiovascular disease and testosterone effects include vasodilation and amelioration of coronary ischemia as well as potentially deleterious effects, it is not possible to predict the net clinical risk-benefit of androgen replacement therapy on cardiovascular disease. Hence, during androgen replacement therapy, it is prudent to aim at maintaining physiologic testosterone concentrations and surveillance of cardiovascular and prostate disease should be comparable with, and no more intensive than, that for eugonadal men of equivalent age (786). The effects of pharmacologic androgen therapy, in which the androgen dose is not necessarily restricted to eugonadal limits, on cardiovascular and prostate disease are still more difficult to predict, and surveillance then depends on the nature, severity, and life expectancy of the underlying disease.
Contraindications and Precautions for Androgen Replacement Therapy
Contraindications to androgen replacement therapy are prostate or breast cancer, because these tumors may be androgen responsive, and pregnancy, in which transplacental passage of androgens may disturb fetal sexual differentiation, notably risking virilization of a female fetus.
The Nobel Prize-winning recognition in the 1940’s that prostate cancer was androgen dependent led to castration being ever since the main treatment for advanced prostate cancer for which it prolongs life but is not curative. This approach led to long-held concern about testosterone treatment of men with advanced prostate cancer (286) for fear of relapse, based however, largely on anecdotal observations (787, 788). Recent studies have challenged this belief as intermittent rather than sustained androgen blockade (789), rapid androgen cycling (790), androgen priming (791, 792) or even testosterone administration (793, 794) have all shown promising, albeit counter-intuitive, results. Meta-analyses suggest that neither ambient circulating testosterone concentrations nor testosterone treatment predict future prostate cancer (454, 455, 795). Furthermore, the increasing diagnosis of organ-confined prostate cancer detected by PSA screening among younger men requires different considerations including the continuation of testosterone replacement therapy following curative treatment of the prostate cancer with careful monitoring (796-798). This is consistent with the fact that endogenous circulating androgens (testosterone, dihydrotestosterone) do not predict subsequent prostate cancer (454) and even prolonged (2 year) administration of high doses of exogenous DHT does not accelerate mid-life prostate growth rate in middle-aged men without prostate disease (681) presumably because exogenous DHT does not increase intra-prostatic androgen concentration (683). Hence local, organ-confined prostate cancer following treatment with curative intent may be an exception to the otherwise absolute contraindication to testosterone for men with a diagnosis of prostate cancer.
Precautions and/or careful monitoring of androgen use are required in (1) initiating treatment in older men with newly diagnosed androgen deficiency who may experience unfamiliar and intolerable initial changes in libido; (2) men subject to occupational monitoring by drug testing (including elite athletes) who may be sanctioned or disqualified for drug use; (3) androgen deficient men with residual spermatogenesis who are planning fertility in the near future who may wish to delay or bank sperm prior to starting treatment; (4) women of reproductive age, especially those who use their voice professionally, who may become irreversibly virilized; (5) prepubertal children in whom inappropriate androgen treatment risks precocious sexual development, virilization and premature epiphyseal closure with compromised final adult height; (6) patients with bleeding disorders or those undergoing anticoagulation or antiplatelet treatment when parenteral administration may cause severe bruising or bleeding; (7) sex steroid–sensitive epilepsy or migraine; and (8) older and especially obese men with subclinical obstructive sleep apnea.
Some traditional warnings about risks of androgen treatment which appear on older product information appear to be rarely or never observed in modern clinical practice. An example of this is hypercalcemia, originally described during pharmacological androgen therapy for advanced breast cancer with metastases (799) although direct causation was not well established (800), but this not been reported with androgen use for other indications. Similarly, fluid overload from sodium and fluid retention due to cardiac or renal failure or severe hypertension is rare and probably confined to high dose pharmacological androgen therapy (799) whereas controlled clinical trials suggest androgens may improve cardiac function and quality of life (394), rather than having detrimental effects, in men with chronic heart failure.
REFERENCES
1. Quigley CA, DeBellis A, Marschke KB, El-Awady MK, Wilson EM, French FF (1995) Androgen receptor defects: historical, clinical and molecular perspectives. Endocrine Reviews 16:271-321
2. Foradori CD, Weiser MJ, Handa RJ (2008) Non-genomic actions of androgens. Frontiers in Neuroendocrinology 29:169-181
3. Michels G, Hoppe UC (2008) Rapid actions of androgens. Frontiers in Neuroendocrinology 29:182-198
4. Gonzalez-Montelongo MC, Marin R, Gomez T, Diaz M (2010) Androgens are powerful non-genomic inducers of calcium sensitization in visceral smooth muscle. Steroids 75:533-538
5. Kochakian CD ed. 1976 Anabolic-Androgenic Steroids. Berlin: Springer-Verlag
6. Nieschlag E, Behre HM eds. 2012 Testosterone: Action.Deficiency.Substitution. 4th ed. Cambridge: Cambridge University Press
7. Hall PF 1988 Testicular steroid synthesis: organization and regulation. In: Knobil E, Neill J eds. The Physiology of Reproduction. New York: Raven Press; 975-998
8. Miller WL, Auchus RJ (2011) The molecular biology, biochemistry, and physiology of human steroidogenesis and its disorders. Endocrine Reviews 32:81-151
9. Neaves WB, Johnson L, Porter JC, Parker CR, Petty CS (1984) Leydig cell numbers, daily sperm production, and serum gonadotropin levels in aging men. Journal of Clinical Endocrinology and Metabolism 55:756-763
10. Miller WL, Tee MK (2015) The post-translational regulation of 17,20 lyase activity. Molecular and Cellular Endocrinology 408:99-106
11. Peng HM, Im SC, Pearl NM, Turcu AF, Rege J, Waskell L, Auchus RJ (2016) Cytochrome b5 Activates the 17,20-Lyase Activity of Human Cytochrome P450 17A1 by Increasing the Coupling of NADPH Consumption to Androgen Production. Biochemistry 55:4356-4365
12. Labrie F (2004) Adrenal androgens and intracrinology. Seminars in Reproductive Medicine 22:299-309
13. Oesterling JE, Epstein JI, Walsh PC (1986) The inability of adrenal androgens to stimulate the adult human prostate: an autopsy evaluation of men with hypogonadotropic hypogonadism and panhypopituitarism. Journal of Urology 136:1030-1034
14. Young J, Couzinet B, Nahoul K, Brailly S, Chanson P, Baulieu EE, Schaison G (1997) Panhypopituitarism as a model to study the metabolism of dehydroepiandrosterone (DHEA) in humans. Journal of Clinical Endocrinology and Metabolism 82:2578-2585
15. Arlt W, Justl HG, Callies F, Reincke M, Hubler D, Oettel M, Ernst M, Schulte HM, Allolio B (1998) Oral dehydroepiandrosterone for adrenal androgen replacement: pharmacokinetics and peripheral conversion to androgens and estrogens in young healthy females after dexamethasone suppression. Journal of Clinical Endocrinology and Metabolism 83:1928-1934
16. Davison SL, Bell R, Donath S, Montalto JG, Davis SR (2005) Androgen levels in adult females: changes with age, menopause, and oophorectomy. Journal of Clinical Endocrinology and Metabolism 90:3847-3853
17. Gallegos AM, Atshaves BP, Storey SM, Starodub O, Petrescu AD, Huang H, McIntosh AL, Martin GG, Chao H, Kier AB, Schroeder F (2001) Gene structure, intracellular localization, and functional roles of sterol carrier protein-2. Progress in Lipid Research 40:498-563
18. Miller WL (2016) Disorders in the initial steps of steroid hormone synthesis. Journal of Steroid Biochemistry and Molecular Biology
19. Midzak A, Zirkin B, Papadopoulos V (2015) Translocator protein: pharmacology and steroidogenesis. Biochemical Society Transactions 43:572-578
20. Jarow JP, Zirkin BR (2005) The androgen microenvironment of the human testis and hormonal control of spermatogenesis. Annals of the New York Academy of Sciences 1061:208-220
21. Gray A, Berlin JA, McKinlay JB, Longcope C (1991) An examination of research design effects on the association of testosterone and male aging: results of a meta-analysis. Journal of Clinical Epidemiology 44:671-684
22. Kaufman JM, Vermeulen A (2005) The decline of androgen levels in elderly men and its clinical and therapeutic implications. Endocrine Reviews 26:833-876
23. Wu FC, Tajar A, Pye SR, Silman AJ, Finn JD, O'Neill TW, Bartfai G, Casanueva F, Forti G, Giwercman A, Huhtaniemi IT, Kula K, Punab M, Boonen S, Vanderschueren D (2008) Hypothalamic-pituitary-testicular axis disruptions in older men are differentially linked to age and modifiable risk factors: the European Male Aging Study. Journal of Clinical Endocrinology and Metabolism 93:2737-2745
24. Sartorius G, Spasevska S, Idan A, Turner L, Forbes E, Zamojska A, Allan C, Ly L, Conway A, McLachlan R, Handelsman D (2012) Serum Testosterone, Dihydrotestosterone and Estradiol Concentrations in Older Men Self-Reporting Very Good Health:The Healthy Man Study. Clinical Endocrinology 77:755-763
25. Handelsman DJ, Staraj S (1985) Testicular size: the effects of aging, malnutrition, and illness. Journal of Andrology 6:144-151
26. Gray A, Feldman HA, McKinlay JB, Longcope C (1991) Age, disease, and changing sex hormone levels in middle-aged men: results of the Massachussetts Male Aging Study. Journal of Clinical Endocrinology and Metabolism 73:1016-1025
27. Feldman HA, Longcope C, Derby CA, Johannes CB, Araujo AB, Coviello AD, Bremner WJ, McKinlay JB (2002) Age trends in the level of serum testosterone and other hormones in middle-aged men: longitudinal results from the Massachusetts male aging study. Journal of Clinical Endocrinology and Metabolism 87:589-598
28. Andersson AM, Jensen TK, Juul A, Petersen JH, Jorgensen T, Skakkebaek NE (2007) Secular decline in male testosterone and sex hormone binding globulin serum levels in Danish population surveys. Journal of Clinical Endocrinology and Metabolism 92:4696-4705
29. Travison TG, Araujo AB, Hall SA, McKinlay JB (2009) Temporal trends in testosterone levels and treatment in older men. Curr Opin Endocrinol Diabetes Obes 16:211-217
30. Perheentupa A, Makinen J, Laatikainen T, Vierula M, Skakkebaek NE, Andersson AM, Toppari J (2013) A cohort effect on serum testosterone levels in Finnish men. European Journal of Endocrinology 168:227-233
31. Taieb J, Mathian B, Millot F, Patricot MC, Mathieu E, Queyrel N, Lacroix I, Somma-Delpero C, Boudou P (2003) Testosterone measured by 10 immunoassays and by isotope-dilution gas chromatography-mass spectrometry in sera from 116 men, women, and children. Clinical Chemistry 49:1381-1395
32. Sikaris K, McLachlan RI, Kazlauskas R, de Kretser D, Holden CA, Handelsman DJ (2005) Reproductive hormone reference intervals for healthy fertile young men: evaluation of automated platform assays. Journal of Clinical Endocrinology and Metabolism 90:5928-5936.
33. Vermeulen A, Desylpere JP, Kaufman JM (1989) Influence of antiopioids on luteinizing hormone pulsatility in aging men. Journal of Clinical Endocrinology and Metabolism 68:68-72
34. Deslypere JP, Kaufman JM, Vermeulen T, Vogelaers D, Vandalem JL, Vermeulen A (1987) Influence of age on pulsatile luteinizing hormone release and responsiveness of the gonadotrophs to sex hormone feedback in men. Journal of Clinical Endocrinology and Metabolism 64:68-73
35. Veldhuis JD, Urban RJ, Lizarralde G, Johnson ML, Iranmanesh A (1992) Attenuation of luteinizing hormone secretory burst amplitude as a proximate basis for the hypoandrogenism of healthy aging men. Journal of Clinical Endocrinology and Metabolism 75:707-713
36. Liu PY, Pincus SM, Takahashi PY, Roebuck PD, Iranmanesh A, Keenan DM, Veldhuis JD (2006) Aging attenuates both the regularity and joint synchrony of LH and testosterone secretion in normal men: analyses via a model of graded GnRH receptor blockade. Am J Physiol Endocrinol Metab 290:E34-E41
37. Mulligan T, Iranmanesh A, Gheorghiu S, Godschalk M, Veldhuis JD (1995) Amplified nocturnal luteinizing hormone (LH) secretory burst frequency with selective attenuation of pulsatile (but not basal) testosterone secretion in healthy aged men: possible Leydig cell desensitization to endogenous LH signaling--a clinical research center study. Journal of Clinical Endocrinology and Metabolism 80:3025-3031
38. Mulligan T, Iranmanesh A, Kerzner R, Demers LW, Veldhuis JD (1999) Two-week pulsatile gonadotropin releasing hormone infusion unmasks dual (hypothalamic and Leydig cell) defects in the healthy aging male gonadotropic axis. European Journal of Endocrinology 141:257-266
39. Liu PY, Takahashi PY, Roebuck PD, Iranmanesh A, Veldhuis JD (2005) Aging in healthy men impairs recombinant human luteinizing hormone (LH)-stimulated testosterone secretion monitored under a two-day intravenous pulsatile LH clamp. Journal of Clinical Endocrinology and Metabolism 90:5544-5550
40. Regadera J, Nistal M, Paniagua R (1985) Testis, epididymis, and spermatic cord in elderly men: correlation of angiographic and histologic studies with systemic arteriosclerosis. Archives of Pathology and Laboratory Medicine 109:663-667
41. Veldhuis JD, Keenan DM, Iranmanesh A (2005) Mechanisms of ensemble failure of the male gonadal axis in aging. Journal of Endocrinological Investigation 28:8-13
42. Veldhuis JD, Keenan DM, Liu PY, Iranmanesh A, Takahashi PY, Nehra AX (2009) The aging male hypothalamic-pituitary-gonadal axis: pulsatility and feedback. Molecular and Cellular Endocrinology 299:14-22
43. Prostate Cancer Trialists' Collaborative Group (2000) Maximum androgen blockade in advanced prostate cancer: an overview of the randomised trials. Lancet 355:1491-1498
44. Arlt W, Callies F, Koehler I, van Vlijmen JC, Fassnacht M, Strasburger CJ, Seibel MJ, Huebler D, Ernst M, Oettel M, Reincke M, Schulte HM, Allolio B (2001) Dehydroepiandrosterone supplementation in healthy men with an age-related decline of dehydroepiandrosterone secretion. Journal of Clinical Endocrinology and Metabolism 86:4686-4692
45. Nair KS, Rizza RA, O'Brien P, Dhatariya K, Short KR, Nehra A, Vittone JL, Klee GG, Basu A, Basu R, Cobelli C, Toffolo G, Dalla Man C, Tindall DJ, Melton LJ, 3rd, Smith GE, Khosla S, Jensen MD (2006) DHEA in elderly women and DHEA or testosterone in elderly men. New England Journal of Medicine 355:1647-1659
46. Baird DT, Horton R, Longcope C, Tait JF (1969) Steroid dynamics under steady-state conditions. Recent Progress in Hormone Research 25:611-664
47. Gurpide E 1975 Tracer Methods in Hormone Research. New York: Springer
48. Setchell BP 1978 The Mammalian Testis. London: Paul Elek
49. Southren AL, Gordon GG, Tochimoto S (1968) Further studies of factors affecting metabolic clearance rate of testosterone in man. Journal of Clinical Endocrinology and Metabolism 28:1105-1112
50. Santner S, Albertson B, Zhang GY, Zhang GH, Santulli M, Wang C, Demers LM, Shackleton C, Santen RJ (1998) Comparative rates of androgen production and metabolism in Caucasian and Chinese subjects. Journal of Clinical Endocrinology and Metabolism 83:2104-2109
51. Wang C, Catlin DH, Starcevic B, Leung A, DiStefano E, Lucas G, Hull L, Swerdloff RS (2004) Testosterone metabolic clearance and production rates determined by stable isotope dilution/tandem mass spectrometry in normal men: influence of ethnicity and age. Journal of Clinical Endocrinology and Metabolism 89:2936-2941
52. Ishimaru T, Edmiston WA, Pages L, Horton R (1978) Splanchnic extraction and conversion of testosterone and dihydrotestosterone in man. Journal of Clinical Endocrinology and Metabolism 46:528-533
53. Longcope C, Sato K, McKay C, Horton R (1984) Aromatization by splanchnic tissue in men. Journal of Clinical Endocrinology and Metabolism 58:1089-1093
54. Diver MJ, Imtiaz KE, Ahmad AM, Vora JP, Fraser WD (2003) Diurnal rhythms of serum total, free and bioavailable testosterone and of SHBG in middle-aged men compared with those in young men. Clinical Endocrinology 58:710-717
55. Bremner WJ, Vitiello MV, Prinz PN (1983) Loss of circadian rhythmicity in blood testosterone levels with aging in normal men. Journal of Clinical Endocrinology and Metabolism 56:1278-1281
56. Keenan DM, Takahashi PY, Liu PY, Roebuck PD, Nehra AX, Iranmanesh A, Veldhuis JD (2006) An ensemble model of the male gonadal axis: illustrative application in aging men. Endocrinology 147:2817-2828
57. Petra P, Stanczyk FZ, Namkung PC, Fritz MA, Novy ML (1985) Direct effect of sex-steroid binding protein (SBP) of plasma on the metabolic clearance rate of testosterone in the rhesus macaque. Journal of Steroid Biochemistry and Molecular Biology 22:739-746
58. Vanbillemont G, Bogaert V, De Bacquer D, Lapauw B, Goemaere S, Toye K, Van Steen K, Taes Y, Kaufman JM (2009) Polymorphisms of the SHBG gene contribute to the interindividual variation of sex steroid hormone blood levels in young, middle-aged and elderly men. Clinical Endocrinology 70:303-310
59. Ohlsson C, Wallaschofski H, Lunetta KL, Stolk L, Perry JR, Koster A, Petersen AK, Eriksson J, Lehtimaki T, Huhtaniemi IT, Hammond GL, Maggio M, Coviello AD, Ferrucci L, Heier M, Hofman A, Holliday KL, Jansson JO, Kahonen M, Karasik D, Karlsson MK, Kiel DP, Liu Y, Ljunggren O, Lorentzon M, Lyytikainen LP, Meitinger T, Mellstrom D, Melzer D, Miljkovic I, Nauck M, Nilsson M, Penninx B, Pye SR, Vasan RS, Reincke M, Rivadeneira F, Tajar A, Teumer A, Uitterlinden AG, Ulloor J, Viikari J, Volker U, Volzke H, Wichmann HE, Wu TS, Zhuang WV, Ziv E, Wu FC, Raitakari O, Eriksson A, Bidlingmaier M, Harris TB, Murray A, de Jong FH, Murabito JM, Bhasin S, Vandenput L, Haring R (2011) Genetic determinants of serum testosterone concentrations in men. PLoS Genet 7:e1002313
60. Jin G, Sun J, Kim ST, Feng J, Wang Z, Tao S, Chen Z, Purcell L, Smith S, Isaacs WB, Rittmaster RS, Zheng SL, Condreay LD, Xu J (2012) Genome-wide association study identifies a new locus JMJD1C at 10q21 that may influence serum androgen levels in men. Human Molecular Genetics 21:5222-5228
61. Xita N, Tsatsoulis A (2010) Genetic variants of sex hormone-binding globulin and their biological consequences. Molecular and Cellular Endocrinology 316:60-65
62. Goldman AL, Bhasin S, Wu FCW, Krishna M, Matsumoto AM, Jasuja R (2017) A Reappraisal of Testosterone's Binding in Circulation: Physiological and Clinical Implications. Endocr Rev 38:302-324
63. Dunn JF, Nisula BC, Rodbard D (1981) Transport of steroid hormones: binding of 21 endogenous steroids to both testosterone-binding globulin and corticosteroid-binding globulin in human plasma. Journal of Clinical Endocrinology and Metabolism 53:58-68
64. Fournier T, Medjoubi NN, Porquet D (2000) Alpha-1-acid glycoprotein. Biochimica et Biophysica Acta 1482:157-171
65. Hammond GL, Wu TS, Simard M (2012) Evolving utility of sex hormone-binding globulin measurements in clinical medicine. Curr Opin Endocrinol Diabetes Obes 19:183-189
66. Wu TS, Hammond GL (2014) Naturally occurring mutants inform SHBG structure and function. Molecular Endocrinology 28:1026-1038
67. Luppa PB, Thaler M, Schulte-Frohlinde E, Schreiegg A, Huber U, Metzger J, Fahrner CL, Hackney AC (2006) Unchanged androgen-binding properties of sex hormone-binding globulin in male patients with liver cirrhosis. Clinical Chemistry and Laboratory Medicine 44:967-973
68. Selva DM, Hammond GL (2006) Human sex hormone-binding globulin is expressed in testicular germ cells and not in sertoli cells. Hormone and Metabolic Research 38:230-235
69. Diaz L, Queipo G, Carino C, Nisembaum A, Larrea F (1997) Biologically active steroid and thyroid hormones stimulate secretion of sex hormone-binding globulin by human term placenta in culture. Archives of Medical Research 28:29-36
70. Handelsman DJ, Conway AJ, Boylan LM (1990) Pharmacokinetics and pharmacodynamics of testosterone pellets in man. Journal of Clinical Endocrinology and Metabolism 71:216-222
71. Wang C, Cunningham G, Dobs A, Iranmanesh A, Matsumoto AM, Snyder PJ, Weber T, Berman N, Hull L, Swerdloff RS (2004) Long-term testosterone gel (AndroGel) treatment maintains beneficial effects on sexual function and mood, lean and fat mass, and bone mineral density in hypogonadal men. Journal of Clinical Endocrinology and Metabolism 89:2085-2098
72. Wittert GA, Harrison RW, Buckley MJ, Wlodarczyk J (2016) An open-label, phase 2, single centre, randomized, crossover design bioequivalence study of AndroForte 5 testosterone cream and Testogel 1% testosterone gel in hypogonadal men: study LP101. Andrology 4:41-45
73. Jaruvongvanich V, Sanguankeo A, Riangwiwat T, Upala S (2017) Testosterone, Sex Hormone-Binding Globulin and Nonalcoholic Fatty Liver Disease: a Systematic Review and Meta-Analysis. Ann Hepatol 16:382-394
74. Sarkar M, VanWagner LB, Terry JG, Carr JJ, Rinella M, Schreiner PJ, Lewis CE, Terrault N (2019) Sex Hormone-Binding Globulin Levels in Young Men Are Associated With Nonalcoholic Fatty Liver Disease in Midlife. Am J Gastroenterol 114:758-763
75. Ahrentsen OD, Jensen HK, Johnsen SG (1982) Sex-hormone-binding globulin deficiency. Lancet 2:377
76. Hogeveen KN, Cousin P, Pugeat M, Dewailly D, Soudan B, Hammond GL (2002) Human sex hormone-binding globulin variants associated with hyperandrogenism and ovarian dysfunction. Journal of Clinical Investigation 109:973-981
77. Vos MJ, Mijnhout GS, Rondeel JM, Baron W, Groeneveld PH (2014) Sex hormone binding globulin deficiency due to a homozygous missense mutation. Journal of Clinical Endocrinology and Metabolism 99:E1798-1802
78. Pardridge WM (1987) Plasma protein-mediated transport of steroid and thyroid hormones. American Journal of Physiology 252:E157-164
79. Mendel CM (1989) The free hormone hypothesis: a physiologically based mathematical model. Endocrine Reviews 10:232-274
80. Ekins R (1990) Measurement of free hormones in blood. Endocrine Reviews 11:5-46
81. Rowland M, Tozer TN 2011 Chapter 17: Drug Interactions. In: Clinical Pharmacokinetics and Pharmacodynamics: Concepts and Applications. 4th ed. Baltimore: Wolters Kluwer/Lippincott Willaims & Wilkins; 483-525
82. Queipo G, Deas M, Arranz C, Carino C, Gonzalez R, Larrea F (1998) Sex hormone-binding globulin stimulates chorionic gonadotrophin secretion from human cytotrophoblasts in culture. Human Reproduction 13:1368-1373
83. Rosner W, Hryb DJ, Khan MS, Nakhla AM, Romas NA (1999) Sex hormone-binding globulin mediates steroid hormone signal transduction at the plasma membrane. Journal of Steroid Biochemistry and Molecular Biology 69:481-485
84. Rosner W, Hryb DJ, Khan MS, Nakhla AM, Romas NA (1999) Androgen and estrogen signaling at the cell membrane via G-proteins and cyclic adenosine monophosphate. Steroids 64:100-106
85. Kahn SM, Li YH, Hryb DJ, Nakhla AM, Romas NA, Cheong J, Rosner W (2008) Sex hormone-binding globulin influences gene expression of LNCaP and MCF-7 cells in response to androgen and estrogen treatment. Advances in Experimental Medicine and Biology 617:557-564
86. Hamada A, Sissung T, Price DK, Danesi R, Chau CH, Sharifi N, Venzon D, Maeda K, Nagao K, Sparreboom A, Mitsuya H, Dahut WL, Figg WD (2008) Effect of SLCO1B3 haplotype on testosterone transport and clinical outcome in caucasian patients with androgen-independent prostatic cancer. Clinical Cancer Research 14:3312-3318
87. Hammes A, Andreassen TK, Spoelgen R, Raila J, Hubner N, Schulz H, Metzger J, Schweigert FJ, Luppa PB, Nykjaer A, Willnow TE (2005) Role of endocytosis in cellular uptake of sex steroids. Cell 122:751-762
88. Adams JS (2005) "Bound" to work: the free hormone hypothesis revisited. Cell 122:647-649
89. Poole CN, Roberts MD, Dalbo VJ, Sunderland KL, Kerksick CM (2011) Megalin and androgen receptor gene expression in young and old human skeletal muscle before and after three sequential exercise bouts. J Strength Cond Res 25:309-317
90. Holt SK, Karyadi DM, Kwon EM, Stanford JL, Nelson PS, Ostrander EA (2008) Association of megalin genetic polymorphisms with prostate cancer risk and prognosis. Clinical Cancer Research 14:3823-3831
91. Handelsman DJ (2017) Free Testosterone: Pumping up the Tires or Ending the Free Ride? Endocr Rev 38:297-301
92. Hsu B, Cumming RG, Blyth FM, Naganathan V, Waite LM, Le Couteur DG, Seibel MJ, Handelsman DJ (2018) Evaluating Calculated Free Testosterone as a Predictor of Morbidity and Mortality Independent of Testosterone for Cross-sectional and 5-Year Longitudinal Health Outcomes in Older Men: The Concord Health and Ageing in Men Project. J Gerontol A Biol Sci Med Sci 73:729-736
93. Sodergard R, Backstrom T, Shanbhag V, Carstensen H (1982) Calculation of free and bound fractions of testosterone and estradiol-17 beta to human plasma proteins at body temperature. Journal of Steroid Biochemistry 16:801-810
94. Vermeulen A, Verdonck L, Kaufman JM (1999) A critical evaluation of simple methods for the estimation of free testosterone in serum. J Clin Endocrinol Metab 84:3666-3672
95. Zakharov MN, Bhasin S, Travison TG, Xue R, Ulloor J, Vasan RS, Carter E, Wu F, Jasuja R (2015) A multi-step, dynamic allosteric model of testosterone's binding to sex hormone binding globulin. Mol Cell Endocrinol 399:190-200
96. Ly LP, Sartorius G, Hull L, Leung A, Swerdloff RS, Wang C, Handelsman DJ (2010) Accuracy of calculated free testosterone formulae in men. Clin Endocrinol (Oxf) 73:382-388
97. Sartorius G, Ly LP, Sikaris K, McLachlan R, Handelsman DJ (2009) Predictive accuracy and sources of variability in calculated free testosterone estimates. Ann Clin Biochem 46:137-143
98. Nanjee MN, Wheeler MJ (1985) Plasma free testosterone--is an index sufficient? Ann Clin Biochem 22 ( Pt 4):387-390
99. Hackbarth JS, Hoyne JB, Grebe SK, Singh RJ (2011) Accuracy of calculated free testosterone differs between equations and depends on gender and SHBG concentration. Steroids 76:48-55
100. Salameh WA, Redor-Goldman MM, Clarke NJ, Reitz RE, Caulfield MP (2010) Validation of a total testosterone assay using high-turbulence liquid chromatography tandem mass spectrometry: total and free testosterone reference ranges. Steroids 75:169-175
101. Ellis GB, Desjardins C (1982) Male rats secrete luteinizing hormone and testosterone episodically. Endocrinology 110:1618-1627
102. Coquelin A, Desjardins C (1982) Luteinizing hormone and testosterone secretion in young and old male mice. American Journal of Physiology 243:E257-263
103. Shackleton C (2010) Clinical steroid mass spectrometry: a 45-year history culminating in HPLC-MS/MS becoming an essential tool for patient diagnosis. Journal of Steroid Biochemistry and Molecular Biology 121:481-490
104. Rosner W, Auchus RJ, Azziz R, Sluss PM, Raff H (2007) Position statement: Utility, limitations, and pitfalls in measuring testosterone: an Endocrine Society position statement. Journal of Clinical Endocrinology and Metabolism 92:405-413
105. Herold DA, Fitzgerald RL (2003) Immunoassays for testosterone in women: better than a guess? Clinical Chemistry 49:1250-1251
106. Umstot ES, Baxter JE, Andersen RN (1985) A theoretically sound and practicable equilibrium dialysis method for measuring percentage of free testosterone. Journal of Steroid Biochemistry 22:639-648
107. Swinkels LM, Ross HA, Benraad TJ (1987) A symmetric dialysis method for the determination of free testosterone in human plasma. Clinica Chimica Acta 165:341-349
108. Hammond GL, Nisker JA, Jones LA, Siiteri PK (1980) Estimation of the percentage of free steroid in undiluted serum by centrifugal ultrafiltration-dialysis. Journal of Biological Chemistry 255:5023-5026
109. Vlahos I, MacMahon W, Sgoutas D, Bowers W, Thompson J, Trawick W (1982) An improved ultrafiltration method for determining free testosterone in serum. Clinical Chemistry 28:2286-2291
110. Vermeulen A, Verdonck L, Kaufman JM (1999) A critical evaluation of simple methods for the estimation of free testosterone in serum. Journal of Clinical Endocrinology and Metabolism 84:3666-3672
111. Rosner W (2001) An extraordinarily inaccurate assay for free testosterone is still with us. Journal of Clinical Endocrinology and Metabolism 86:2903.
112. Fritz KS, McKean AJ, Nelson JC, Wilcox RB (2008) Analog-based free testosterone test results linked to total testosterone concentrations, not free testosterone concentrations. Clinical Chemistry 54:512-516
113. Kapoor P, Luttrell BM, Williams D (1993) The free androgen index is not valid for adult males. Journal of Steroid Biochemistry and Molecular Biology 45:325-326
114. Mazer NA (2009) A novel spreadsheet method for calculating the free serum concentrations of testosterone, dihydrotestosterone, estradiol, estrone and cortisol: with illustrative examples from male and female populations. Steroids 74:512-519
115. Ly LP, Handelsman DJ (2005) Empirical estimation of free testosterone from testosterone and sex hormone-binding globulin immunoassays. European Journal of Endocrinology 152:471-478
116. Ly LP, Sartorius G, Hull L, Leung A, Swerdloff RS, Wang C, Handelsman DJ (2010) Accuracy of calculated free testosterone formulae in men. Clinical Endocrinology 73:382-388
117. Sartorius G, Ly LP, Sikaris K, McLachlan R, Handelsman DJ (2009) Predictive accuracy and sources of variability in calculated free testosterone estimates. Annals of Clinical Biochemistry 46:137-143
118. Egleston BL, Chandler DW, Dorgan JF (2010) Validity of estimating non-sex hormone-binding globulin bound testosterone and oestradiol from total hormone measurements in boys and girls. Annals of Clinical Biochemistry 47:233-241
119. Giton F, Fiet J, Guechot J, Ibrahim F, Bronsard F, Chopin D, Raynaud JP (2006) Serum bioavailable testosterone: assayed or calculated? Clinical Chemistry 52:474-481
120. Van Eenoo P, Delbeke FT (2006) Metabolism and excretion of anabolic steroids in doping control--new steroids and new insights. Journal of Steroid Biochemistry and Molecular Biology 101:161-178
121. Kumar N, Crozat A, Li F, Catterall JF, Bardin CW, Sundaram K (1999) 7alpha-methyl-19-nortestosterone, a synthetic androgen with high potency: structure-activity comparisons with other androgens. Journal of Steroid Biochemistry and Molecular Biology 71:213-222
122. Deslypere JP, Young M, Wilson JD, McPhaul MJ (1992) Testosterone and 5 alpha-dihydrotestosterone interact differently with the androgen receptor to enhance transcription of the MMTV-CAT reporter gene. Molecular and Cellular Endocrinology 88:15-22
123. Zhou ZX, Lane MV, Kemppainen JA, French FS, Wilson EM (1995) Specificity of ligand-dependent androgen receptor stabilization: receptor domain interactions influence ligand dissociation and receptor stability. Molecular Endocrinology 9:208-218
124. McRobb L, Handelsman DJ, Kazlauskas R, Wilkinson S, McLeod MD, Heather AK (2008) Structure-activity relationships of synthetic progestins in a yeast-based in vitro androgen bioassay. Journal of Steroid Biochemistry and Molecular Biology 110:39-47
125. Russell DW, Wilson JD (1994) Steroid 5 alpha-reductase: two genes/two enzymes. Annual Review of Biochemistry 63:25-61
126. Thigpen AE, Davis DL, Milatovich A, Mendonca B, Imperato-McGinley J, Griffin JE, Francke U, Wilson JD, Russell DW (1992) Molecular genetics of steroid 5a-reductase 2 deficiency. Journal of Clinical Investigations 90:799-809
127. Cai LQ, Fratiani CM, Gautier T, Imperato-McGinley J (1994) Dihydrotestosterone regulation of semen in males pseudohermaphrodites with 5-a reductase deficiency. Journal of Clinical Endocrinology and Metabolism 79:409-414
128. Sobel V, Schwartz B, Zhu YS, Cordero JJ, Imperato-McGinley J (2006) Bone mineral density in the complete androgen insensitivity and 5alpha-reductase-2 deficiency syndromes. Journal of Clinical Endocrinology and Metabolism 91:3017-3023
129. Imperato-McGinley J, Peterson RE, Gautier T, Sturla E (1979) Androgens and the evolution of male gender identity among male pseudohemaphrodites with 5-a reductase deficiency. New England Journal of Medicine 300:1233-1237
130. Imperato-McGinley J, Gautier T, Zirinsky K, Hom T, Palomo O, Stein E, Vaughan ED, Markisz JA, deArellano ER, Kazam E (1992) Prostate visualization studies in males homozygous and heterozygous for 5-a reductase deficiency. Journal of Clinical Endocrinology and Metabolism 75:1022-1026
131. Imperato-McGinley J, Zhu YS (2002) Androgens and male physiology the syndrome of 5alpha-reductase-2 deficiency. Molecular and Cellular Endocrinology 198:51-59
132. Steers WD (2001) 5alpha-reductase activity in the prostate. Urology 58:17-24; discussion 24.
133. Frick J, Aulitzky W (1991) Physiology of the prostate. Infection 19 Suppl 3:S115-118
134. Gisleskog PO, Hermann D, Hammarlund-Udenaes M, Karlsson MO (1998) A model for the turnover of dihydrotestosterone in the presence of the irreversible 5 alpha-reductase inhibitors GI198745 and finasteride. Clinical Pharmacology and Therapeutics 64:636-647
135. Miller LR, Partin AW, Chan DW, Bruzek DJ, Dobs AS, Epstein JI, Walsh PC (1998) Influence of radical prostatectomy on serum hormone levels. Journal of Urology 160:449-453
136. Toorians AW, Kelleher S, Gooren LJ, Jimenez M, Handelsman DJ (2003) Estimating the contribution of the prostate to blood dihydrotestosterone. Journal of Clinical Endocrinology and Metabolism 88:5207-5211
137. Zhu YS, Katz MD, Imperato-McGinley J (1998) Natural potent androgens: lessons from human genetic models. Baillieres Clinical Endocrinology and Metabolism 12:83-113
138. Uemura M, Tamura K, Chung S, Honma S, Okuyama A, Nakamura Y, Nakagawa H (2008) Novel 5 alpha-steroid reductase (SRD5A3, type-3) is overexpressed in hormone-refractory prostate cancer. Cancer Sci 99:81-86
139. Cantagrel V, Lefeber DJ, Ng BG, Guan Z, Silhavy JL, Bielas SL, Lehle L, Hombauer H, Adamowicz M, Swiezewska E, De Brouwer AP, Blumel P, Sykut-Cegielska J, Houliston S, Swistun D, Ali BR, Dobyns WB, Babovic-Vuksanovic D, van Bokhoven H, Wevers RA, Raetz CR, Freeze HH, Morava E, Al-Gazali L, Gleeson JG (2010) SRD5A3 is required for converting polyprenol to dolichol and is mutated in a congenital glycosylation disorder. Cell 142:203-217
140. Thompson IM, Goodman PJ, Tangen CM, Lucia MS, Miller GJ, Ford LG, Lieber MM, Cespedes RD, Atkins JN, Lippman SM, Carlin SM, Ryan A, Szczepanek CM, Crowley JJ, Coltman CA, Jr. (2003) The influence of finasteride on the development of prostate cancer. New England Journal of Medicine 349:215-224
141. Andriole GL, Bostwick DG, Brawley OW, Gomella LG, Marberger M, Montorsi F, Pettaway CA, Tammela TL, Teloken C, Tindall DJ, Somerville MC, Wilson TH, Fowler IL, Rittmaster RS (2010) Effect of dutasteride on the risk of prostate cancer. New England Journal of Medicine 362:1192-1202
142. Lucia MS, Epstein JI, Goodman PJ, Darke AK, Reuter VE, Civantos F, Tangen CM, Parnes HL, Lippman SM, La Rosa FG, Kattan MW, Crawford ED, Ford LG, Coltman CA, Jr., Thompson IM (2007) Finasteride and high-grade prostate cancer in the Prostate Cancer Prevention Trial. Journal of the National Cancer Institute 99:1375-1383
143. Redman MW, Tangen CM, Goodman PJ, Lucia MS, Coltman CA, Jr., Thompson IM (2008) Finasteride does not increase the risk of high-grade prostate cancer: a bias-adjusted modeling approach. Cancer Prev Res (Phila) 1:174-181
144. Kim J, Amos CI, Logothetis C (2011) 5alpha-Reductase inhibitors for prostate-cancer prevention. New England Journal of Medicine 365:2340
145. Simpson ER, Zhao Y, Agarwal VR, Michael MD, Bulun SE, Hinshelwood MM, Graham-Lorence S, Sun T, Fisher CR, Qin K, Mendelson CR (1997) Aromatase expression in health and disease. Recent Progress in Hormone Research 52:185-213; discussion 213-184
146. Bulun SE, Takayama K, Suzuki T, Sasano H, Yilmaz B, Sebastian S (2004) Organization of the human aromatase p450 (CYP19) gene. Seminars in Reproductive Medicine 22:5-9
147. Naftolin F (1994) Brain aromatization of androgens. Journal of Reproductive Medicine 39:257-261
148. Roselli CF (2007) Brain aromatase: roles in reproduction and neuroprotection. Journal of Steroid Biochemistry and Molecular Biology 106:143-150
149. Jones ME, Boon WC, Proietto J, Simpson ER (2006) Of mice and men: the evolving phenotype of aromatase deficiency. Trends Endocrinol Metab 17:55-64
150. Lubahn DB, Moyer JS, Golding TS, Couse JF, Korach KS, Smithies O (1993) Alteration of reproductive function but not prenatal sexual development after insertional disruption of the mouse estrogen receptor gene. Proceedings of the National Academy of Sciences USA 90:11162-11166
151. Couse JE, Mahato D, Eddy EM, Korach KS (2001) Molecular mechanism of estrogen action in the male: insights from the estrogen receptor null mice. Reproduction, Fertility, and Development 13:211-219
152. Gennari L, Nuti R, Bilezikian JP (2004) Aromatase activity and bone homeostasis in men. Journal of Clinical Endocrinology and Metabolism 89:5898-5907
153. Vanderschueren D, Vandenput L, Boonen S, Lindberg MK, Bouillon R, Ohlsson C (2004) Androgens and bone. Endocrine Reviews 25:389-425
154. Vandenput L, Swinnen JV, Boonen S, Van Herck E, Erben RG, Bouillon R, Vanderschueren D (2004) Role of the androgen receptor in skeletal homeostasis: the androgen-resistant testicular feminized male mouse model. Journal of Bone and Mineral Research 19:1462-1470
155. Chesnut CH, 3rd, Ivey JL, Gruber HE, Matthews M, Nelp WB, Sisom K, Baylink DJ (1983) Stanozolol in postmenopausal osteoporosis: therapeutic efficacy and possible mechanisms of action. Metabolism: Clinical and Experimental 32:571-580
156. Need AG, Nordin BEC, Chatterton BE (1993) Double-blind placebo-controlled trial of treatment of osteoporosis with the anabolic steroid nandrolone decanoate. Osteoporosis International 3 Suppl 1:S218-222
157. Venken K, De Gendt K, Boonen S, Ophoff J, Bouillon R, Swinnen JV, Verhoeven G, Vanderschueren D (2006) Relative impact of androgen and estrogen receptor activation in the effects of androgens on trabecular and cortical bone in growing male mice: a study in the androgen receptor knockout mouse model. Journal of Bone and Mineral Research 21:576-585
158. Callewaert F, Venken K, Ophoff J, De Gendt K, Torcasio A, van Lenthe GH, Van Oosterwyck H, Boonen S, Bouillon R, Verhoeven G, Vanderschueren D (2009) Differential regulation of bone and body composition in male mice with combined inactivation of androgen and estrogen receptor-alpha. FASEB Journal 23:232-240
159. Zuloaga DG, Puts DA, Jordan CL, Breedlove SM (2008) The role of androgen receptors in the masculinization of brain and behavior: what we've learned from the testicular feminization mutation. Hormones and Behavior 53:613-626
160. Finkelstein JS, Lee H, Burnett-Bowie SA, Pallais JC, Yu EW, Borges LF, Jones BF, Barry CV, Wulczyn KE, Thomas BJ, Leder BZ (2013) Gonadal steroids and body composition, strength, and sexual function in men. New England Journal of Medicine 369:1011-1022
161. Sartorius GA, Ly LP, Handelsman DJ (2014) Male sexual function can be maintained without aromatization: randomized placebo-controlled trial of dihydrotestosterone (DHT) in healthy, older men for 24 months. J Sex Med 11:2562-2570
162. Vanderschueren D, Gaytant J, Boonen S, Venken K (2008) Androgens and bone. Curr Opin Endocrinol Diabetes Obes 15:250-254
163. Zhou SF (2008) Drugs behave as substrates, inhibitors and inducers of human cytochrome P450 3A4. Current Drug Metabolism 9:310-322
164. Chouinard S, Yueh MF, Tukey RH, Giton F, Fiet J, Pelletier G, Barbier O, Belanger A (2008) Inactivation by UDP-glucuronosyltransferase enzymes: the end of androgen signaling. Journal of Steroid Biochemistry and Molecular Biology 109:247-253
165. Jakobsson J, Ekstrom L, Inotsume N, Garle M, Lorentzon M, Ohlsson C, Roh HK, Carlstrom K, Rane A (2006) Large differences in testosterone excretion in Korean and Swedish men are strongly associated with a UDP-glucuronosyl transferase 2B17 polymorphism. Journal of Clinical Endocrinology and Metabolism 91:687-693
166. Schulze JJ, Lundmark J, Garle M, Skilving I, Ekstrom L, Rane A (2008) Doping test results dependent on genotype of uridine diphospho-glucuronosyl transferase 2B17, the major enzyme for testosterone glucuronidation. Journal of Clinical Endocrinology and Metabolism 93:2500-2506
167. Johnsen SG, Bennet EP, Jensen VG (1974) Therapeutic effectiveness of oral testosterone. Lancet ii:1473-1475
168. Frey H, Aakvag A, Saanum D, Falch J (1979) Bioavailability of testosterone in males. European Journal of Clinical Pharmacology 16:345-349
169. Parkes AS (1938) Effective absorption of hormones. British Medical Journal:371-373
170. Lisser H, Escamilla RF, Curtis LE (1942) Testosterone therapy of male eunuchoids. III Sublingual administration of testosterone compounds. Journal of Clinical Endocrinology 2:351-360
171. Korbonits M, Slawik M, Cullen D, Ross RJ, Stalla G, Schneider H, Reincke M, Bouloux PM, Grossman AB (2004) A comparison of a novel testosterone bioadhesive buccal system, striant, with a testosterone adhesive patch in hypogonadal males. Journal of Clinical Endocrinology and Metabolism 89:2039-2043
172. Wang C, Eyre DR, Clark R, Kleinberg D, Newman C, Iranmanesh A, Veldhuis J, Dudley RE, Berman N, Davidson T, Barstow TJ, Sinow R, Alexander G, Swerdloff RS (1996) Sublingual testosterone replacement improves muscle mass and strength, decreases bone resorption, and increases bone formation markers in hypogonadal men--a clinical research center study. Journal of Clinical Endocrinology and Metabolism 81:3654-3662
173. Shackleford DM, Faassen WA, Houwing N, Lass H, Edwards GA, Porter CJ, Charman WN (2003) Contribution of lymphatically transported testosterone undecanoate to the systemic exposure of testosterone after oral administration of two andriol formulations in conscious lymph duct-cannulated dogs. Journal of Pharmacology and Experimental Therapeutics 306:925-933
174. Foss GL (1939) Clinical administration of androgens. Lancet i:502-504
175. Bousfield GR, Butnev VY, Gotschall RR, Baker VL, Moore WT (1996) Structural features of mammalian gonadotropins. Molecular and Cellular Endocrinology 125:3-19
176. Gromoll J, Eiholzer U, Nieschlag E, Simoni M (2000) Male hypogonadism caused by homozygous deletion of exon 10 of the luteinizing hormone (LH) receptor: differential action of human chorionic gonadotropin and LH. Journal of Clinical Endocrinology and Metabolism 85:2281-2286
177. O'Shaughnessy PJ, Baker P, Sohnius U, Haavisto AM, Charlton HM, Huhtaniemi I (1998) Fetal development of Leydig cell activity in the mouse is independent of pituitary gonadotroph function. Endocrinology 139:1141-1146
178. Abreu AP, Dauber A, Macedo DB, Noel SD, Brito VN, Gill JC, Cukier P, Thompson IR, Navarro VM, Gagliardi PC, Rodrigues T, Kochi C, Longui CA, Beckers D, de Zegher F, Montenegro LR, Mendonca BB, Carroll RS, Hirschhorn JN, Latronico AC, Kaiser UB (2013) Central precocious puberty caused by mutations in the imprinted gene MKRN3. N Engl J Med 368:2467-2475
179. Dauber A, Cunha-Silva M, Macedo DB, Brito VN, Abreu AP, Roberts SA, Montenegro LR, Andrew M, Kirby A, Weirauch MT, Labilloy G, Bessa DS, Carroll RS, Jacobs DC, Chappell PE, Mendonca BB, Haig D, Kaiser UB, Latronico AC (2017) Paternally Inherited DLK1 Deletion Associated With Familial Central Precocious Puberty. J Clin Endocrinol Metab 102:1557-1567
180. Roberts S, Kaiser UB (2020) GENETICS IN ENDOCRINOLOGY: Genetic etiologies of central precocious puberty and the role of imprinted genes. Eur J Endocrinol
181. Howard SR (2019) The Genetic Basis of Delayed Puberty. Front Endocrinol (Lausanne) 10:423
182. Meikle AW, Bishop DT, Stringham JD, West DW (1986) Quantitating genetic and nongenetic factors that determine plasma sex steroid variation in normal male twins. Metabolism 35:1090-1095
183. Eaves L, Silberg J, Foley D, Bulik C, Maes H, Erkanli A, Angold A, Costello EJ, Worthman C (2004) Genetic and environmental influences on the relative timing of pubertal change. Twin Res 7:471-481
184. Grotzinger AD, Mann FD, Patterson MW, Herzhoff K, Tackett JL, Tucker-Drob EM, Paige Harden K (2018) Twin models of environmental and genetic influences on pubertal development, salivary testosterone, and estradiol in adolescence. Clin Endocrinol (Oxf) 88:243-250
185. Silventoinen K, Haukka J, Dunkel L, Tynelius P, Rasmussen F (2008) Genetics of pubertal timing and its associations with relative weight in childhood and adult height: the Swedish Young Male Twins Study. Pediatrics 121:e885-891
186. Day FR, Perry JR, Ong KK (2015) Genetic Regulation of Puberty Timing in Humans. Neuroendocrinology 102:247-255
187. Busch AS, Hollis B, Day FR, Sørensen K, Aksglaede L, Perry JRB, Ong KK, Juul A, Hagen CP (2019) Voice break in boys-temporal relations with other pubertal milestones and likely causal effects of BMI. Hum Reprod 34:1514-1522
188. Sultan C, Gaspari L, Maimoun L, Kalfa N, Paris F (2018) Disorders of puberty. Best Pract Res Clin Obstet Gynaecol 48:62-89
189. Sisk CL, Foster DL (2004) The neural basis of puberty and adolescence. Nature Neuroscience 7:1040-1047
190. Abreu AP, Kaiser UB (2016) Pubertal development and regulation. Lancet Diabetes Endocrinol 4:254-264
191. Veldhuis JD (2003) Neuroendocrine facets of human puberty. Neurobiology of Aging 24 Suppl 1:S93-S119; discussion S121-112
192. Terasawa E, Fernandez DL (2001) Neurobiological mechanisms of the onset of puberty in primates. Endocrine Reviews 22:111-151
193. de Roux N, Genin E, Carel JC, Matsuda F, Chaussain JL, Milgrom E (2003) Hypogonadotropic hypogonadism due to loss of function of the KiSS1-derived peptide receptor GPR54. Proceedings of the National Academy of Sciences of the United States of America 100:10972-10976
194. Seminara SB, Messager S, Chatzidaki EE, Thresher RR, Acierno JS, Jr., Shagoury JK, Bo-Abbas Y, Kuohung W, Schwinof KM, Hendrick AG, Zahn D, Dixon J, Kaiser UB, Slaugenhaupt SA, Gusella JF, O'Rahilly S, Carlton MB, Crowley WF, Jr., Aparicio SA, Colledge WH (2003) The GPR54 gene as a regulator of puberty. New England Journal of Medicine 349:1614-1627
195. Lomniczi A, Ojeda SR (2016) The Emerging Role of Epigenetics in the Regulation of Female Puberty. Endocrine Development 29:1-16
196. Wu FC, Borrow SM, Nicol K, Elton R, Hunter WM (1989) Ontogeny of pulsatile gonadotrophin secretion and pituitary responsiveness in male puberty in man: a mixed longitudinal and cross-sectional study. Journal of Endocrinology 123:347-359
197. Pedersen-White JR, Chorich LP, Bick DP, Sherins RJ, Layman LC (2008) The prevalence of intragenic deletions in patients with idiopathic hypogonadotropic hypogonadism and Kallmann syndrome. Molecular Human Reproduction 14:367-370
198. Delemarre-van de Waal HA (2005) Secular trend of timing of puberty. Endocrine Development 8:1-14
199. Ong KK, Ahmed ML, Dunger DB (2006) Lessons from large population studies on timing and tempo of puberty (secular trends and relation to body size): the European trend. Molecular and Cellular Endocrinology 254-255:8-12
200. Parent AS, Teilmann G, Juul A, Skakkebaek NE, Toppari J, Bourguignon JP (2003) The timing of normal puberty and the age limits of sexual precocity: variations around the world, secular trends, and changes after migration. Endocrine Reviews 24:668-693
201. Gluckman PD, Hanson MA (2006) Changing times: the evolution of puberty. Molecular and Cellular Endocrinology 254-255:26-31
202. Slyper AH (2006) The pubertal timing controversy in the USA, and a review of possible causative factors for the advance in timing of onset of puberty. Clinical Endocrinology 65:1-8
203. Juul A, Teilmann G, Scheike T, Hertel NT, Holm K, Laursen EM, Main KM, Skakkebaek NE (2006) Pubertal development in Danish children: comparison of recent European and US data. International Journal of Andrology 29:247-255; discussion 286-290
204. Day FR, Elks CE, Murray A, Ong KK, Perry JR (2015) Puberty timing associated with diabetes, cardiovascular disease and also diverse health outcomes in men and women: the UK Biobank study. Sci Rep 5:11208
205. Day FR, Bulik-Sullivan B, Hinds DA, Finucane HK, Murabito JM, Tung JY, Ong KK, Perry JR (2015) Shared genetic aetiology of puberty timing between sexes and with health-related outcomes. Nat Commun 6:8842
206. Veldhuis JD, Keenan DM, Pincus SM (2010) Regulation of complex pulsatile and rhythmic neuroendocrine systems: the male gonadal axis as a prototype. Progress in Brain Research 181:79-110
207. Chin WW, Boime I eds. 1990 Glycoprotein Hormones: Structure, Synthesis and Biologic Function. Norwell: Serono Symposia, USA
208. Boime I, Ben-Menahem D (1999) Glycoprotein hormone structure-function and analog design. Recent Progress in Hormone Research 54:271-288; discussion 288-279
209. Ascoli M, Fanelli F, Segaloff DL (2002) The lutropin/choriogonadotropin receptor, a 2002 perspective. Endocrine Reviews 23:141-174
210. Rosa C, Amr S, Birken S, Wehmann R, Nisula B (1984) Effect of desialylation of human chorionic gonadotropin on its metabolic clearance rate in humans. Journal of Clinical Endocrinology and Metabolism 59:1215-1219
211. Muyan M, Furuhashi M, Sugahara T, Boime I (1996) The carboxy-terminal region of the beta-subunits of luteinizing hormone and chorionic gonadotropin differentially influence secretion and assembly of the heterodimers. Molecular Endocrinology 10:1678-1687
212. Bouloux PM, Handelsman DJ, Jockenhovel F, Nieschlag E, Rabinovici J, Frasa WL, de Bie JJ, Voortman G, Itskovitz-Eldor J (2001) First human exposure to FSH-CTP in hypogonadotrophic hypogonadal males. Human Reproduction 16:1592-1597.
213. Joshi L, Murata Y, Wondisford FE, Szkudlinski MW, Desai R, Weintraub BD (1995) Recombinant thyrotropin containing a beta-subunit chimera with the human chorionic gonadotropin-beta carboxy-terminus is biologically active, with a prolonged plasma half-life: role of carbohydrate in bioactivity and metabolic clearance. Endocrinology 136:3839-3848
214. Fares F, Ganem S, Hajouj T, Agai E (2007) Development of a long-acting erythropoietin by fusing the carboxyl-terminal peptide of human chorionic gonadotropin beta-subunit to the coding sequence of human erythropoietin. Endocrinology 148:5081-5087
215. Saunders PT (2003) Germ cell-somatic cell interactions during spermatogenesis. Reproduction Supplement 61:91-101
216. Rudolfsson SH, Wikstrom P, Jonsson A, Collin O, Bergh A (2004) Hormonal regulation and functional role of vascular endothelial growth factor a in the rat testis. Biology of Reproduction 70:340-347
217. Schnorr JA, Bray MJ, Veldhuis JD (2001) Aromatization mediates testosterone's short-term feedback restraint of 24-hour endogenously driven and acute exogenous gonadotropin-releasing hormone-stimulated luteinizing hormone and follicle-stimulating hormone secretion in young men. Journal of Clinical Endocrinology and Metabolism 86:2600-2606
218. Pitteloud N, Dwyer AA, DeCruz S, Lee H, Boepple PA, Crowley WF, Jr., Hayes FJ (2008) Inhibition of luteinizing hormone secretion by testosterone in men requires aromatization for its pituitary but not its hypothalamic effects: evidence from the tandem study of normal and gonadotropin-releasing hormone-deficient men. Journal of Clinical Endocrinology and Metabolism 93:784-791
219. Wilson JD (2001) The role of 5alpha-reduction in steroid hormone physiology. Reproduction, Fertility, and Development 13:673-678
220. Simpson ER (2004) Aromatase: biologic relevance of tissue-specific expression. Seminars in Reproductive Medicine 22:11-23
221. Cooke PS, Nanjappa MK, Ko C, Prins GS, Hess RA (2017) Estrogens in Male Physiology. Physiol Rev 97:995-1043
222. Naftolin F, Ryan KJ, Petro Z (1971) Aromatization of androstenedione by the diencephalon. J Clin Endocrinol Metab 33:368-370
223. Smith EP, Boyd J, Frank GR, Takahashi H, Cohen RM, Specker B, Williams TC, Lubahn DB, Korach KS (1994) Estrogen resistance caused by a mutation in the estrogen-receptor gene in a man. N Engl J Med 331:1056-1061
224. Krege JH, Hodgin JB, Couse JF, Enmark E, Warner M, Mahler JF, Sar M, Korach KS, Gustafsson JA, Smithies O (1998) Generation and reproductive phenotypes of mice lacking estrogen receptor beta. Proc Natl Acad Sci U S A 95:15677-15682
225. Finkelstein JS, Lee H, Burnett-Bowie SA, Pallais JC, Yu EW, Borges LF, Jones BF, Barry CV, Wulczyn KE, Thomas BJ, Leder BZ (2013) Gonadal steroids and body composition, strength, and sexual function in men. N Engl J Med 369:1011-1022
226. Vanderschueren D, Laurent MR, Claessens F, Gielen E, Lagerquist MK, Vandenput L, Borjesson AE, Ohlsson C (2014) Sex steroid actions in male bone. Endocr Rev 35:906-960
227. Russell N, Grossmann M (2019) MECHANISMS IN ENDOCRINOLOGY: Estradiol as a male hormone. Eur J Endocrinol 181:R23-R43
228. Gronemeyer H, Gustafsson JA, Laudet V (2004) Principles for modulation of the nuclear receptor superfamily. Nature Reviews Drug Discovery 3:950-964
229. Shi Y (2007) Orphan nuclear receptors in drug discovery. Drug Discov Today 12:440-445
230. Adachi M, Takayanagi R, Tomura A, Imasaki K, Kato S, Goto K, Yanase T, Ikuyama S, Nawata H (2000) Androgen-insensitivity syndrome as a possible coactivator disease. New England Journal of Medicine 343:856-862
231. McEwan IJ, Lavery D, Fischer K, Watt K (2007) Natural disordered sequences in the amino terminal domain of nuclear receptors: lessons from the androgen and glucocorticoid receptors. Nucl Recept Signal 5:e001
232. Rajender S, Singh L, Thangaraj K (2007) Phenotypic heterogeneity of mutations in androgen receptor gene. Asian Journal of Andrology 9:147-179
233. Zitzmann M, Nieschlag E (2003) The CAG repeat polymorphism within the androgen receptor gene and maleness. International Journal of Andrology 26:76-83.
234. Simanainen U, Brogley M, Gao YR, Jimenez M, Harwood DT, Handelsman DJ, Robins DM (2011) Length of the human androgen receptor glutamine tract determines androgen sensitivity in vivo. Molecular and Cellular Endocrinology 342:81-86
235. Zitzmann M, Depenbusch M, Gromoll J, Nieschlag E (2003) Prostate volume and growth in testosterone-substituted hypogonadal men are dependent on the CAG repeat polymorphism of the androgen receptor gene: a longitudinal pharmacogenetic study. Journal of Clinical Endocrinology and Metabolism 88:2049-2054
236. Zitzmann M, Nieschlag E (2007) Androgen receptor gene CAG repeat length and body mass index modulate the safety of long-term intramuscular testosterone undecanoate therapy in hypogonadal men. Journal of Clinical Endocrinology and Metabolism 92:3844-3853
237. Zeegers MP, Kiemeney LA, Nieder AM, Ostrer H (2004) How strong is the association between CAG and GGN repeat length polymorphisms in the androgen receptor gene and prostate cancer risk? Cancer Epidemiology, Biomarkers and Prevention 13:1765-1771
238. Davis-Dao CA, Tuazon ED, Sokol RZ, Cortessis VK (2007) Male infertility and variation in CAG repeat length in the androgen receptor gene: a meta-analysis. Journal of Clinical Endocrinology and Metabolism 92:4319-4326
239. Ioannidis JP, Ntzani EE, Trikalinos TA, Contopoulos-Ioannidis DG (2001) Replication validity of genetic association studies. Nature Genetics 29:306-309
240. Palazzolo I, Gliozzi A, Rusmini P, Sau D, Crippa V, Simonini F, Onesto E, Bolzoni E, Poletti A (2008) The role of the polyglutamine tract in androgen receptor. Journal of Steroid Biochemistry and Molecular Biology 108:245-253
241. Thomas PS, Jr., Fraley GS, Damian V, Woodke LB, Zapata F, Sopher BL, Plymate SR, La Spada AR (2006) Loss of endogenous androgen receptor protein accelerates motor neuron degeneration and accentuates androgen insensitivity in a mouse model of X-linked spinal and bulbar muscular atrophy. Human Molecular Genetics 15:2225-2238
242. Atsuta N, Watanabe H, Ito M, Banno H, Suzuki K, Katsuno M, Tanaka F, Tamakoshi A, Sobue G (2006) Natural history of spinal and bulbar muscular atrophy (SBMA): a study of 223 Japanese patients. Brain 129:1446-1455
243. Adachi H, Waza M, Katsuno M, Tanaka F, Doyu M, Sobue G (2007) Pathogenesis and molecular targeted therapy of spinal and bulbar muscular atrophy. Neuropathology and Applied Neurobiology 33:135-151
244. Ross CA, Poirier MA (2005) Opinion: What is the role of protein aggregation in neurodegeneration? Nature Reviews Molecular Cell Biology 6:891-898
245. Palazzolo I, Stack C, Kong L, Musaro A, Adachi H, Katsuno M, Sobue G, Taylor JP, Sumner CJ, Fischbeck KH, Pennuto M (2009) Overexpression of IGF-1 in muscle attenuates disease in a mouse model of spinal and bulbar muscular atrophy. Neuron 63:316-328
246. Rinaldi C, Bott LC, Chen KL, Harmison GG, Katsuno M, Sobue G, Pennuto M, Fischbeck KH (2012) IGF-1 administration ameliorates disease manifestations in a mouse model of spinal and bulbar muscular atrophy. Molecular Medicine
247. Katsuno M, Banno H, Suzuki K, Takeuchi Y, Kawashima M, Yabe I, Sasaki H, Aoki M, Morita M, Nakano I, Kanai K, Ito S, Ishikawa K, Mizusawa H, Yamamoto T, Tsuji S, Hasegawa K, Shimohata T, Nishizawa M, Miyajima H, Kanda F, Watanabe Y, Nakashima K, Tsujino A, Yamashita T, Uchino M, Fujimoto Y, Tanaka F, Sobue G (2010) Efficacy and safety of leuprorelin in patients with spinal and bulbar muscular atrophy (JASMITT study): a multicentre, randomised, double-blind, placebo-controlled trial. Lancet Neurol 9:875-884
248. Banno H, Katsuno M, Suzuki K, Tanaka F, Sobue G (2012) Pathogenesis and molecular targeted therapy of spinal and bulbar muscular atrophy (SBMA). Cell and Tissue Research 349:313-320
249. Grunseich C, Fischbeck KH (2015) Spinal and Bulbar Muscular Atrophy. Neurologic Clinics 33:847-854
250. Prescott J, Coetzee GA (2006) Molecular chaperones throughout the life cycle of the androgen receptor. Cancer Letters 231:12-19
251. Heinlein CA, Chang C (2002) Androgen receptor (AR) coregulators: an overview. Endocrine Reviews 23:175-200
252. Smith CL, O'Malley BW (2004) Coregulator function: a key to understanding tissue specificity of selective receptor modulators. Endocrine Reviews 25:45-71
253. Gottlieb B, Beitel LK, Nadarajah A, Paliouras M, Trifiro M (2012) The androgen receptor gene mutations database: 2012 update. Human Mutation 33:887-894
254. Antonarakis ES, Lu C, Wang H, Luber B, Nakazawa M, Roeser JC, Chen Y, Mohammad TA, Chen Y, Fedor HL, Lotan TL, Zheng Q, De Marzo AM, Isaacs JT, Isaacs WB, Nadal R, Paller CJ, Denmeade SR, Carducci MA, Eisenberger MA, Luo J (2014) AR-V7 and resistance to enzalutamide and abiraterone in prostate cancer. New England Journal of Medicine 371:1028-1038
255. McCrea E, Sissung TM, Price DK, Chau CH, Figg WD (2016) Androgen receptor variation affects prostate cancer progression and drug resistance. Pharmacological Research 114:152-162
256. Sarpel U, Palmer SK, Dolgin SE (2005) The incidence of complete androgen insensitivity in girls with inguinal hernias and assessment of screening by vaginal length measurement. Journal of Pediatric Surgery 40:133-136; discussion 136-137
257. Hiort O, Holterhus PM, Horter T, Schulze W, Kremke B, Bals-Pratsch M, Sinnecker GH, Kruse K (2000) Significance of mutations in the androgen receptor gene in males with idiopathic infertility. Journal of Clinical Endocrinology and Metabolism 85:2810-2815
258. Belgorosky A, Rivarola MA (1985) Sex hormone binding globulin response to testosterone. An androgen sensitivity test. Acta Endocrinologica 109:130-138
259. Sinnecker GH, Hiort O, Nitsche EM, Holterhus PM, Kruse K (1997) Functional assessment and clinical classification of androgen sensitivity in patients with mutations of the androgen receptor gene. German Collaborative Intersex Study Group. European Journal of Pediatrics 156:7-14
260. Cheikhelard A, Morel Y, Thibaud E, Lortat-Jacob S, Jaubert F, Polak M, Nihoul-Fekete C (2008) Long-term followup and comparison between genotype and phenotype in 29 cases of complete androgen insensitivity syndrome. Journal of Urology 180:1496-1501
261. Marcus R, Leary D, Schneider DL, Shane E, Favus M, Quigley CA (2000) The contribution of testosterone to skeletal development and maintenance: lessons from the androgen insensitivity syndrome. Journal of Clinical Endocrinology and Metabolism 85:1032-1037
262. Danilovic DL, Correa PH, Costa EM, Melo KF, Mendonca BB, Arnhold IJ (2007) Height and bone mineral density in androgen insensitivity syndrome with mutations in the androgen receptor gene. Osteoporosis International 18:369-374
263. Han TS, Goswami D, Trikudanathan S, Creighton SM, Conway GS (2008) Comparison of bone mineral density and body proportions between women with complete androgen insensitivity syndrome and women with gonadal dysgenesis. European Journal of Endocrinology 159:179-185
264. Wisniewski AB, Migeon CJ, Meyer-Bahlburg HF, Gearhart JP, Berkovitz GD, Brown TR, Money J (2000) Complete androgen insensitivity syndrome: long-term medical, surgical, and psychosexual outcome. Journal of Clinical Endocrinology and Metabolism 85:2664-2669
265. Hines M, Ahmed SF, Hughes IA (2003) Psychological outcomes and gender-related development in complete androgen insensitivity syndrome. Archives of Sexual Behavior 32:93-101
266. Minto CL, Liao KL, Conway GS, Creighton SM (2003) Sexual function in women with complete androgen insensitivity syndrome. Fertility and Sterility 80:157-164
267. Brinkmann L, Schuetzmann K, Richter-Appelt H (2007) Gender assignment and medical history of individuals with different forms of intersexuality: evaluation of medical records and the patients' perspective. J Sex Med 4:964-980
268. Hughes IA, Werner R, Bunch T, Hiort O (2012) Androgen insensitivity syndrome. Seminars in Reproductive Medicine 30:432-442
269. Ahmed SF, Cheng A, Dovey L, Hawkins JR, Martin H, Rowland J, Shimura N, Tait AD, Hughes IA (2000) Phenotypic features, androgen receptor binding, and mutational analysis in 278 clinical cases reported as androgen insensitivity syndrome. Journal of Clinical Endocrinology and Metabolism 85:658-665
270. Deeb A, Mason C, Lee YS, Hughes IA (2005) Correlation between genotype, phenotype and sex of rearing in 111 patients with partial androgen insensitivity syndrome. Clinical Endocrinology 63:56-62
271. Migeon CJ, Wisniewski AB, Gearhart JP, Meyer-Bahlburg HF, Rock JA, Brown TR, Casella SJ, Maret A, Ngai KM, Money J, Berkovitz GD (2002) Ambiguous genitalia with perineoscrotal hypospadias in 46,XY individuals: long-term medical, surgical, and psychosexual outcome. Pediatrics 110:e31
272. Gottlieb B, Beitel LK, Wu JH, Trifiro M (2004) The androgen receptor gene mutations database (ARDB): 2004 update. Human Mutation 23:527-533
273. Rodien P, Mebarki F, Mowszowicz I, Chaussain JL, Young J, Morel Y, Schaison G (1996) Different phenotypes in a family with androgen insensitivity caused by the same M780I point mutation in the androgen receptor gene. Journal of Clinical Endocrinology and Metabolism 81:2994-2998
274. Boehmer AL, Brinkmann O, Bruggenwirth H, van Assendelft C, Otten BJ, Verleun-Mooijman MC, Niermeijer MF, Brunner HG, Rouwe CW, Waelkens JJ, Oostdijk W, Kleijer WJ, van der Kwast TH, de Vroede MA, Drop SL (2001) Genotype versus phenotype in families with androgen insensitivity syndrome. Journal of Clinical Endocrinology and Metabolism 86:4151-4160
275. Kohler B, Lumbroso S, Leger J, Audran F, Grau ES, Kurtz F, Pinto G, Salerno M, Semitcheva T, Czernichow P, Sultan C (2005) Androgen insensitivity syndrome: somatic mosaicism of the androgen receptor in seven families and consequences for sex assignment and genetic counseling. Journal of Clinical Endocrinology and Metabolism 90:106-111
276. Boehmer AL, Brinkmann AO, Nijman RM, Verleun-Mooijman MC, de Ruiter P, Niermeijer MF, Drop SL (2001) Phenotypic variation in a family with partial androgen insensitivity syndrome explained by differences in 5alpha dihydrotestosterone availability. Journal of Clinical Endocrinology and Metabolism 86:1240-1246
277. MacLean HE, Favaloro JM, Warne GL, Zajac JD (2006) Double-strand DNA break repair with replication slippage on two strands: a novel mechanism of deletion formation. Human Mutation 27:483-489
278. Rogowski W (2006) Genetic screening by DNA technology: a systematic review of health economic evidence. International Journal of Technology Assessment in Health Care 22:327-337
279. Hiort O, Sinnecker GH, Holterhus PM, Nitsche EM, Kruse K (1998) Inherited and de novo androgen receptor gene mutations: investigation of single-case families. Journal of Pediatrics 132:939-943
280. Yeh SH, Chiu CM, Chen CL, Lu SF, Hsu HC, Chen DS, Chen PJ (2007) Somatic mutations at the trinucleotide repeats of androgen receptor gene in male hepatocellular carcinoma. International Journal of Cancer 120:1610-1617
281. Hiort O, Naber SP, Lehners A, Muletta-Feurer S, Sinnecker GH, Zollner A, Komminoth P (1996) The role of androgen receptor gene mutations in male breast carcinoma. Journal of Clinical Endocrinology and Metabolism 81:3404-3407
282. Paul R, Breul J (2000) Antiandrogen withdrawal syndrome associated with prostate cancer therapies: incidence and clinical significance. Drug Safety 23:381-390
283. Suzuki H, Okihara K, Miyake H, Fujisawa M, Miyoshi S, Matsumoto T, Fujii M, Takihana Y, Usui T, Matsuda T, Ozono S, Kumon H, Ichikawa T, Miki T (2008) Alternative nonsteroidal antiandrogen therapy for advanced prostate cancer that relapsed after initial maximum androgen blockade. Journal of Urology 180:921-927
284. Hara T, Miyazaki J, Araki H, Yamaoka M, Kanzaki N, Kusaka M, Miyamoto M (2003) Novel mutations of androgen receptor: a possible mechanism of bicalutamide withdrawal syndrome. Cancer Research 63:149-153
285. Miyamoto H, Rahman MM, Chang C (2004) Molecular basis for the antiandrogen withdrawal syndrome. Journal of Cellular Biochemistry 91:3-12
286. Huggins C, Hodges CV (1941) Studies on prostatic cancer. I. The effect of castration, of estrogen and of androgen injection on serum phosphatases in metastatic carcinoma of the prostate. Cancer Research 1:293-297
287. Labrie F, Cusan L, Gomez JL, Martel C, Berube R, Belanger P, Belanger A, Vandenput L, Mellstrom D, Ohlsson C (2008) Comparable amounts of sex steroids are made outside the gonads in men and women: Strong lesson for hormone therapy of prostate and breast cancer. Journal of Steroid Biochemistry and Molecular Biology
288. Attard G, Reid AH, Yap TA, Raynaud F, Dowsett M, Settatree S, Barrett M, Parker C, Martins V, Folkerd E, Clark J, Cooper CS, Kaye SB, Dearnaley D, Lee G, de Bono JS (2008) Phase I clinical trial of a selective inhibitor of CYP17, abiraterone acetate, confirms that castration-resistant prostate cancer commonly remains hormone driven. Journal of Clinical Oncology 26:4563-4571
289. Anonymous (2000) Maximum androgen blockade in advanced prostate cancer: an overview of the randomised trials. Prostate Cancer Trialists' Collaborative Group. Lancet 355:1491-1498
290. Pezaro CJ, Mukherji D, De Bono JS (2012) Abiraterone acetate: redefining hormone treatment for advanced prostate cancer. Drug Discov Today 17:221-226
291. Scher HI, Fizazi K, Saad F, Taplin ME, Sternberg CN, Miller K, de Wit R, Mulders P, Chi KN, Shore ND, Armstrong AJ, Flaig TW, Flechon A, Mainwaring P, Fleming M, Hainsworth JD, Hirmand M, Selby B, Seely L, de Bono JS, Investigators A (2012) Increased survival with enzalutamide in prostate cancer after chemotherapy. New England Journal of Medicine 367:1187-1197
292. Meikle AW (2004) The interrelationships between thyroid dysfunction and hypogonadism in men and boys. Thyroid 14 Suppl 1:S17-25
293. Payne KL, Loidl NM, Lim CF, Topliss DJ, Stockigt JR, Barlow JW (1997) Modulation of T3-induced sex hormone-binding globulin secretion by human hepatoblastoma cells. European Journal of Endocrinology 137:415-420
294. Isojarvi J (2008) Disorders of reproduction in patients with epilepsy: antiepileptic drug related mechanisms. Seizure 17:111-119
295. Wheeler MJ, Toone BK, ADannatt, Fenwick PB, Brown S (1991) Metabolic clearance rate of testosterone in male epileptic patients on anti-convulsant therapy. Journal of Endocrinology 129:465-468
296. Death AK, McGrath KC, Handelsman DJ (2005) Valproate is an anti-androgen and anti-progestin. Steroids 70:946-953
297. Green JRB, Goble HL, Edwards CRW, Dawson AM (1977) Reversible insensitivity to androgens in men with untreated gluten enteropathy. Lancet i:280-282
298. Farthing MJR, Rees LH, Edwards CRW, Dawson AM (1983) Male gonadal dysfunction in coeliac disease: 2. Sex hormones. Gut 24:127-135
299. Frydman M, Kauschansky A, Bonne-Tamir B, Nassar F, Homburg R (1991) Assessment of the hypothalamic-pituitary-testicular function in male patients with Wilson's disease. Journal of Andrology 12:180-184
300. Herrick AL, McColl KE, Wallace AM, Moore MR, Goldberg A (1990) Elevation of hormone-binding globulins in acute intermittent porphyria. Clinica Chimica Acta 187:141-148
301. Iturriaga H, Lioi X, Valladares L (1999) Sex hormone-binding globulin in non-cirrhotic alcoholic patients during early withdrawal and after longer abstinence. Alcohol and Alcoholism 34:903-909
302. Handelsman DJ, Strasser S, McDonald JA, Conway AJ, McCaughan GW (1995) Hypothalamic-pituitary testicular function in end-stage non-alcoholic liver disease before and after liver transplantation. Clinical Endocrinology 43:331-337
303. Hamilton JB, Mestler GE (1969) Mortality and survival: comparison of eunuchs with intact men and women in a mentally retarded population. Journal of Gerontology 24:395-411
304. Nieschlag E, Nieschlag S, Behre HM (1993) Lifespan and testosterone. Nature 366:215
305. Jenkins JS (1998) The voice of the castrato. Lancet 351:1877-1880
306. Eyben FE, Graugaard C, Vaeth M (2005) All-cause mortality and mortality of myocardial infarction for 989 legally castrated men. European Journal of Epidemiology 20:863-869
307. Min KJ, Lee CK, Park HN (2012) The lifespan of Korean eunuchs. Current Biology 22:R792-793
308. Bojesen A, Juul S, Birkebaek N, Gravholt CH (2004) Increased mortality in Klinefelter syndrome. Journal of Clinical Endocrinology and Metabolism 89:3830-3834
309. Liu PY, Death AK, Handelsman DJ (2003) Androgens and cardiovascular disease. Endocrine Reviews 24:313-340
310. Liu PY, Handelsman DJ 2004 Androgen therapy in non-gonadal disease. In: Nieschlag E, Behre HM eds. Testosterone: Action, Deficiency and Substitution. 3rd ed. Berlin: Springer-Verlag; 445-495
311. Zuraw BL (2008) Clinical practice. Hereditary angioedema. New England Journal of Medicine 359:1027-1036
312. Longhurst H, Cicardi M (2012) Hereditary angio-oedema. Lancet 379:474-481
313. Handelsman DJ (2007) Update in andrology. Journal of Clinical Endocrinology and Metabolism 92:4505-4511
314. Bojesen A, Juul S, Gravholt CH (2003) Prenatal and postnatal prevalence of Klinefelter syndrome: a national registry study. Journal of Clinical Endocrinology and Metabolism 88:622-626
315. Kelleher S, Conway AJ, Handelsman DJ (2004) Blood testosterone threshold for androgen deficiency symptoms. Journal of Clinical Endocrinology and Metabolism 89:3813-3817
316. Schaison G, Young J, Pholsena M, Nahoul K, Couzinet B (1993) Failure of combined follicle-stimulating hormone-testosterone administration to initiate and/or maintain spermatogenesis in men with hypogonadotropic hypogonadism. Journal of Clinical Endocrinology and Metabolism 77:1545-1549
317. Pitteloud N, Hayes FJ, Dwyer A, Boepple PA, Lee H, Crowley WF, Jr. (2002) Predictors of outcome of long-term GnRH therapy in men with idiopathic hypogonadotropic hypogonadism. Journal of Clinical Endocrinology and Metabolism 87:4128-4136
318. Wang C, Tso SC, Todd D (1989) Hypogonadotropic hypogonadism in severe beta-thalassemia: effect of chelation and pulsatile gonadotropin-releasing hormone therapy. Journal of Clinical Endocrinology and Metabolism 68:511-516
319. Liu PY, Baker HW, Jayadev V, Zacharin M, Conway AJ, Handelsman DJ (2009) Induction of spermatogenesis and fertility during gonadotropin treatment of gonadotropin-deficient infertile men: predictors of fertility outcome. Journal of Clinical Endocrinology and Metabolism 94:801-808
320. Dwyer AA, Raivio T, Pitteloud N (2015) Gonadotrophin replacement for induction of fertility in hypogonadal men. Best Practice and Research Clinical Endocrinology and Metabolism 29:91-103
321. Handelsman DJ, Goebel C, Idan A, Jimenez M, Trout G, Kazlauskas R (2009) Effects of recombinant human LH and hCG on serum and urine LH and androgens in men. Clinical Endocrinology 71:417-428
322. Barrio R, de Luis D, Alonso M, Lamas A, Moreno JC (1999) Induction of puberty with human chorionic gonadotropin and follicle-stimulating hormone in adolescent males with hypogonadotropic hypogonadism. Fertility and Sterility 71:244-248
323. Bouvattier C, Maione L, Bouligand J, Dode C, Guiochon-Mantel A, Young J (2011) Neonatal gonadotropin therapy in male congenital hypogonadotropic hypogonadism. Nat Rev Endocrinol 8:172-182
324. Howard S, Dunkel L (2016) Sex Steroid and Gonadotropin Treatment in Male Delayed Puberty. Endocrine Development 29:185-197
325. Belanger A, Candas B, Dupont A, Cusan L, Diamond P, Gomez JL, Labrie F (1994) Changes in serum concentrations of conjugated and unconjugated steroids in 40- to 80-year-old men. Journal of Clinical Endocrinology and Metabolism 79:1086-1090
326. Orwoll E, Lambert LC, Marshall LM, Phipps K, Blank J, Barrett-Connor E, Cauley J, Ensrud K, Cummings S (2006) Testosterone and estradiol among older men. Journal of Clinical Endocrinology and Metabolism 91:1336-1344
327. Travison TG, Araujo AB, Kupelian V, O'Donnell AB, McKinlay JB (2007) The relative contributions of aging, health, and lifestyle factors to serum testosterone decline in men. Journal of Clinical Endocrinology and Metabolism 92:549-555
328. Andersson AM, Jorgensen N, Frydelund-Larsen L, Rajpert-De Meyts E, Skakkebaek NE (2004) Impaired Leydig cell function in infertile men: a study of 357 idiopathic infertile men and 318 proven fertile controls. Journal of Clinical Endocrinology and Metabolism 89:3161-3167
329. Howell SJ, Radford JA, Adams JE, Shalet SM (2000) The impact of mild Leydig cell dysfunction following cytotoxic chemotherapy on bone mineral density (BMD) and body composition. Clinical Endocrinology 52:609-616
330. Howell SJ, Radford JA, Smets EM, Shalet SM (2000) Fatigue, sexual function and mood following treatment for haematological malignancy: the impact of mild Leydig cell dysfunction. British Journal of Cancer 82:789-793
331. Gerl A, Muhlbayer D, Hansmann G, Mraz W, Hiddemann W (2001) The impact of chemotherapy on Leydig cell function in long term survivors of germ cell tumors. Cancer 91:1297-1303
332. Somali M, Mpatakoias V, Avramides A, Sakellari I, Kaloyannidis P, Smias C, Anagnostopoulos A, Kourtis A, Rousso D, Panidis D, Vagenakis A (2005) Function of the hypothalamic-pituitary-gonadal axis in long-term survivors of hematopoietic stem cell transplantation for hematological diseases. Gynecological Endocrinology 21:18-26
333. Howell SJ, Radford JA, Adams JE, Smets EM, Warburton R, Shalet SM (2001) Randomized placebo-controlled trial of testosterone replacement in men with mild Leydig cell insufficiency following cytotoxic chemotherapy. Clinical Endocrinology 55:315-324
334. Merza Z, Blumsohn A, Mah PM, Meads DM, McKenna SP, Wylie K, Eastell R, Wu F, Ross RJ (2006) Double-blind placebo-controlled study of testosterone patch therapy on bone turnover in men with borderline hypogonadism. International Journal of Andrology 29:381-391
335. Nieschlag E (2010) Male hormonal contraception. Handb Exp Pharmacol:197-223
336. Handelsman DJ 2011 Androgen therapy in non-gonadal disease. In: Nieschlag E, Behre HM eds. Testosterone: Action, Deficiency and Substitution. 4th ed. Cambridge: Cambridge University Press; 372-407
337. Teruel JL, Aguilera A, Marcen R, Antolin JN, Otero GG, Ortuno J (1996) Androgen therapy for anaemia of chronic renal failure. Scandanavian Journal of Urology and Nephrology 30:403-408
338. Gascon A, Belvis JJ, Berisa F, Iglesias E, Estopinan V, Teruel JL (1999) Nandrolone decanoate is a good alternative for the treatment of anemia in elderly male patients on hemodialysis. Geriatric Nephrology and Urology 9:67-72
339. Navarro JF, Mora C, Macia M, Garcia J (2002) Randomized prospective comparison between erythropoietin and androgens in CAPD patients. Kidney International 61:1537-1544.
340. Ishak KG, Zimmerman HJ (1987) Hepatotoxic effects of the anabolic-androgenic steroids. Seminars in Liver Disease 7:230-236
341. Velazquez I, Alter BP (2004) Androgens and liver tumors: Fanconi's anemia and non-Fanconi's conditions. American Journal of Hematology 77:257-267
342. Sloane DE, Lee CW, Sheffer AL (2007) Hereditary angioedema: Safety of long-term stanozolol therapy. Journal of Allergy and Clinical Immunology 120:654-658
343. Banerji A, Sloane DE, Sheffer AL (2008) Hereditary angioedema: a current state-of-the-art review, V: attenuated androgens for the treatment of hereditary angioedema. Annals of Allergy, Asthma, and Immunology 100:S19-22
344. Bork K, Bygum A, Hardt J (2008) Benefits and risks of danazol in hereditary angioedema: a long-term survey of 118 patients. Annals of Allergy, Asthma, and Immunology 100:153-161
345. Sabharwal G, Craig T (2015) Recombinant human C1 esterase inhibitor for the treatment of hereditary angioedema due to C1 inhibitor deficiency (C1-INH-HAE). Expert Rev Clin Immunol 11:319-327
346. Bhasin S, Storer TW, Berman N, Callegari C, Clevenger B, Phillips J, Bunnell TJ, Tricker R, Shirazi A, Casaburi R (1996) The effects of supraphysiologic doses of testosterone on muscle size and strength in normal men. New England Journal of Medicine 335:1-7
347. Elashoff JD, Jacknow AD, Shain SG, Braunstein GD (1991) Effects of anabolic-androgenic steroids on muscular strength. Annals of Internal Medicine 115:387-393
348. Storer TW, Magliano L, Woodhouse L, Lee ML, Dzekov C, Dzekov J, Casaburi R, Bhasin S (2003) Testosterone dose-dependently increases maximal voluntary strength and leg power, but does not affect fatigability or specific tension. Journal of Clinical Endocrinology and Metabolism 88:1478-1485
349. Storer TW, Woodhouse L, Magliano L, Singh AB, Dzekov C, Dzekov J, Bhasin S (2008) Changes in muscle mass, muscle strength, and power but not physical function are related to testosterone dose in healthy older men. Journal of the American Geriatrics Society
350. Bhasin S, Woodhouse L, Casaburi R, Singh AB, Mac RP, Lee M, Yarasheski KE, Sinha-Hikim I, Dzekov C, Dzekov J, Magliano L, Storer TW (2005) Older men are as responsive as young men to the anabolic effects of graded doses of testosterone on the skeletal muscle. Journal of Clinical Endocrinology and Metabolism 90:678-688
351. Coviello AD, Lakshman K, Mazer NA, Bhasin S (2006) Differences in the apparent metabolic clearance rate of testosterone in young and older men with gonadotropin suppression receiving graded doses of testosterone. Journal of Clinical Endocrinology and Metabolism 91:4669-4675
352. Coviello AD, Kaplan B, Lakshman KM, Chen T, Singh AB, Bhasin S (2008) Effects of graded doses of testosterone on erythropoiesis in healthy young and older men. Journal of Clinical Endocrinology and Metabolism 93:914-919
353. Singh AB, Hsia S, Alaupovic P, Sinha-Hikim I, Woodhouse L, Buchanan TA, Shen R, Bross R, Berman N, Bhasin S (2002) The effects of varying doses of T on insulin sensitivity, plasma lipids, apolipoproteins, and C-reactive protein in healthy young men. Journal of Clinical Endocrinology and Metabolism 87:136-143
354. Gray PB, Singh AB, Woodhouse LJ, Storer TW, Casaburi R, Dzekov J, Dzekov C, Sinha-Hikim I, Bhasin S (2005) Dose-dependent effects of testosterone on sexual function, mood and visuospatial cognition in older men. Journal of Clinical Endocrinology and Metabolism 90:3838-3846
355. Basaria S, Coviello AD, Travison TG, Storer TW, Farwell WR, Jette AM, Eder R, Tennstedt S, Ulloor J, Zhang A, Choong K, Lakshman KM, Mazer NA, Miciek R, Krasnoff J, Elmi A, Knapp PE, Brooks B, Appleman E, Aggarwal S, Bhasin G, Hede-Brierley L, Bhatia A, Collins L, LeBrasseur N, Fiore LD, Bhasin S (2010) Adverse events associated with testosterone administration. The New England Journal of Medicine 363:109-122
356. Kotler DP, Tierney AR, Wang J, Pierson RN (1989) Magnitude of body-cell-mass depletion and the timing of death from wasting in AIDS. American Journal of Clinical Nutrition 50:444-447
357. Moyle GJ, Schoelles K, Fahrbach K, Frame D, James K, Scheye R, Cure-Bolt N (2004) Efficacy of selected treatments of HIV wasting: a systematic review and meta-analysis. Journal of Acquired Immune Deficiency Syndromes 37 Suppl 5:S262-276
358. Johns K, Beddall MJ, Corrin RC (2005) Anabolic steroids for the treatment of weight loss in HIV-infected individuals. Cochrane Database Syst Rev:CD005483
359. Bolding G, Sherr L, Maguire M, Elford J (1999) HIV risk behaviours among gay men who use anabolic steroids. Addiction 94:1829-1835
360. Lambert CP, Sullivan DH, Freeling SA, Lindquist DM, Evans WJ (2002) Effects of testosterone replacement and/or resistance exercise on the composition of megestrol acetate stimulated weight gain in elderly men: a randomized controlled trial. Journal of Clinical Endocrinology and Metabolism 87:2100-2106
361. Mulligan K, Zackin R, Von Roenn JH, Chesney MA, Egorin MJ, Sattler FR, Benson CA, Liu T, Umbleja T, Shriver S, Auchus RJ, Schambelan M (2007) Testosterone supplementation of megestrol therapy does not enhance lean tissue accrual in men with human immunodeficiency virus-associated weight loss: a randomized, double-blind, placebo-controlled, multicenter trial. Journal of Clinical Endocrinology and Metabolism 92:563-570
362. Vuong C, Van Uum SH, O'Dell LE, Lutfy K, Friedman TC (2010) The effects of opioids and opioid analogs on animal and human endocrine systems. Endocrine Reviews 31:98-132
363. Bawor M, Bami H, Dennis BB, Plater C, Worster A, Varenbut M, Daiter J, Marsh DC, Steiner M, Anglin R, Coote M, Pare G, Thabane L, Samaan Z (2015) Testosterone suppression in opioid users: a systematic review and meta-analysis. Drug and Alcohol Dependence 149:1-9
364. O'Rourke TK, Jr., Wosnitzer MS (2016) Opioid-Induced Androgen Deficiency (OPIAD): Diagnosis, Management, and Literature Review. Current Urology Reports 17:76
365. Daniell HW, Lentz R, Mazer NA (2006) Open-label pilot study of testosterone patch therapy in men with opioid-induced androgen deficiency. J Pain 7:200-210
366. Basaria S, Travison TG, Alford D, Knapp PE, Teeter K, Cahalan C, Eder R, Lakshman K, Bachman E, Mensing G, Martel MO, Le D, Stroh H, Bhasin S, Wasan AD, Edwards RR (2015) Effects of testosterone replacement in men with opioid-induced androgen deficiency: a randomized controlled trial. Pain 156:280-288
367. Huang G, Travison TG, Edwards RR, Basaria S (2016) Effects of Testosterone Replacement on Pain Catastrophizing and Sleep Quality in Men with Opioid-Induced Androgen Deficiency. Pain Med
368. Wierman ME, Basson R, Davis SR, Khosla S, Miller KK, Rosner W, Santoro N (2006) Androgen therapy in women: an Endocrine Society Clinical Practice guideline. Journal of Clinical Endocrinology and Metabolism 91:3697-3710
369. Arlt W, Callies F, van Vlijmen JC, Koehler I, Reincke M, Bidlingmaier M, Huebler D, Oettel M, Ernst M, Schulte HM, Allolio B (1999) Dehydroepiandrosterone replacement in women with adrenal insufficiency New England Journal of Medicine 341:1013-1020
370. Gurnell EM, Hunt PJ, Curran SE, Conway CL, Pullenayegum EM, Huppert FA, Compston JE, Herbert J, Chatterjee VK (2008) Long-term DHEA replacement in primary adrenal insufficiency: a randomized, controlled trial. Journal of Clinical Endocrinology and Metabolism 93:400-409
371. Johannsson G, Burman P, Wiren L, Engstrom BE, Nilsson AG, Ottosson M, Jonsson B, Bengtsson BA, Karlsson FA (2002) Low dose dehydroepiandrosterone affects behavior in hypopituitary androgen-deficient women: a placebo-controlled trial. Journal of Clinical Endocrinology and Metabolism 87:2046-2052
372. Lovas K, Gebre-Medhin G, Trovik TS, Fougner KJ, Uhlving S, Nedrebo BG, Myking OL, Kampe O, Husebye ES (2003) Replacement of dehydroepiandrosterone in adrenal failure: no benefit for subjective health status and sexuality in a 9-month, randomized, parallel group clinical trial. Journal of Clinical Endocrinology and Metabolism 88:1112-1118
373. Miller KK, Biller BM, Beauregard C, Lipman JG, Jones J, Schoenfeld D, Sherman JC, Swearingen B, Loeffler J, Klibanski A (2006) Effects of testosterone replacement in androgen-deficient women with hypopituitarism: a randomized, double-blind, placebo-controlled study. Journal of Clinical Endocrinology and Metabolism 91:1683-1690
374. Shifren JL, Braunstein GD, Simon JA, Casson PR, Buster JE, Redmond GP, Burki RE, Ginsburg ES, Rosen RC, Leiblum SR, Caramelli KE, Mazer NA (2000) Transdermal testosterone treatment in women with impaired sexual function after oophorectomy. New England Journal of Medicine 343:682-688
375. Davis S, Papalia MA, Norman RJ, O'Neill S, Redelman M, Williamson M, Stuckey BG, Wlodarczyk J, Gard'ner K, Humberstone A (2008) Safety and efficacy of a testosterone metered-dose transdermal spray for treating decreased sexual satisfaction in premenopausal women: a randomized trial. Annals of Internal Medicine 148:569-577
376. Somboonporn W, Davis S, Seif MW, Bell R (2005) Testosterone for peri- and postmenopausal women. Cochrane Database Syst Rev:CD004509
377. Greenblatt RB, Barfield WE, Garner JF, Calk GL, Harrod JP (1950) Evaluation of an estrogen, androgen, estrogen-androgen combination, and a placebo in the treatment of the menopause. Journal of Clinical Endocrinology and Metabolism 10:1547-1558
378. Sherwin BB, Gelfand MM (1987) The role of androgens in the maintenance of sexual functioning in oophorectomized women. Psychosomatic Medicine 49:397-409
379. Urman B, Pride SM, Yuen BH (1991) Elevated serum testosterone, hirsutism, and virilism associated with combined androgen-estrogen hormone replacement therapy. Obstetrics and Gynecology 77:1124-1131
380. Gerritsma EJ, Brocaar MP, Hakkesteegt MM, Birkenhager JC (1994) Virilization of the voice in post-menopausal women due to the anabolic steroid nandrolone decanoate (Decadurabolin). The effects of medication for one year. Clin Otolaryngol Allied Sci 19:79-84
381. Baker J (1999) A report on alterations to the speaking and singing voices of four women following hormonal therapy with virilizing agents. Journal of Voice 13:496-507
382. Davis SR, McCloud P, Strauss BJG, Burger H (1995) Testosterone enhances estradiol's effects on postmenopausal bone density and sexuality. Maturitas 21:227-236
383. Elraiyah T, Sonbol MB, Wang Z, Khairalseed T, Asi N, Undavalli C, Nabhan M, Firwana B, Altayar O, Prokop L, Montori VM, Murad MH (2014) Clinical review: The benefits and harms of systemic testosterone therapy in postmenopausal women with normal adrenal function: a systematic review and meta-analysis. Journal of Clinical Endocrinology and Metabolism 99:3543-3550
384. Davis SR, Wahlin-Jacobsen S (2015) Testosterone in women--the clinical significance. Lancet Diabetes Endocrinol 3:980-992
385. Reed BG, Bou Nemer L, Carr BR (2016) Has testosterone passed the test in premenopausal women with low libido? A systematic review. Int J Womens Health 8:599-607
386. Miller KK, Grieco KA, Klibanski A (2005) Testosterone administration in women with anorexia nervosa. Journal of Clinical Endocrinology and Metabolism 90:1428-1433
387. Choi HH, Gray PB, Storer TW, Calof OM, Woodhouse L, Singh AB, Padero C, Mac RP, Sinha-Hikim I, Shen R, Dzekov J, Dzekov C, Kushnir MM, Rockwood AL, Meikle AW, Lee ML, Hays RD, Bhasin S (2005) Effects of testosterone replacement in human immunodeficiency virus-infected women with weight loss. Journal of Clinical Endocrinology and Metabolism 90:1531-1541
388. Gordon C, Wallace DJ, Shinada S, Kalunian KC, Forbess L, Braunstein GD, Weisman MH (2008) Testosterone patches in the management of patients with mild/moderate systemic lupus erythematosus. Rheumatology 47:334-338
389. Ferreira IM, Verreschi IT, Nery LE, Goldstein RS, Zamel N, Brooks D, Jardim JR (1998) The influence of 6 months of oral anabolic steroids on body mass and respiratory muscles in undernourished COPD patients. Chest 114:19-28.
390. Casaburi R, Bhasin S, Cosentino L, Porszasz J, Somfay A, Lewis MI, Fournier M, Storer TW (2004) Effects of testosterone and resistance training in men with chronic obstructive pulmonary disease. American Journal of Respiratory and Critical Care Medicine 170:870-878
391. Svartberg J, Aasebo U, Hjalmarsen A, Sundsfjord J, Jorde R (2004) Testosterone treatment improves body composition and sexual function in men with COPD, in a 6-month randomized controlled trial. Respiratory Medicine 98:906-913
392. Weisberg J, Wanger J, Olson J, Streit B, Fogarty C, Martin T, Casaburi R (2002) Megestrol acetate stimulates weight gain and ventilation in underweight COPD patients. Chest 121:1070-1078
393. Jankowska EA, Biel B, Majda J, Szklarska A, Lopuszanska M, Medras M, Anker SD, Banasiak W, Poole-Wilson PA, Ponikowski P (2006) Anabolic deficiency in men with chronic heart failure: prevalence and detrimental impact on survival. Circulation 114:1829-1837
394. Malkin CJ, Pugh PJ, West JN, van Beek EJ, Jones TH, Channer KS (2006) Testosterone therapy in men with moderate severity heart failure: a double-blind randomized placebo controlled trial. European Heart Journal 27:57-64
395. Handelsman DJ, Dong Q 1992 Ontogenic regression: a model of stress and reproduction. In: Sheppard K, Boublik JH, Funder JW eds. Stress and Reproduction. New York: Raven Press; 333-345
396. Reid IR, Wattie DJ, Evans MC, Stapleton JP (1996) Testosterone therapy in glucocorticoid-treated men. Archives of Internal Medicine 156:1173-1177
397. Crawford BA, Liu PY, Kean M, Bleasel J, Handelsman DJ (2003) Randomised, placebo-controlled trial of androgen effects on bone and muscle in men requiring long-term systemic glucocorticoid therapy. Journal of Clinical Endocrinology and Metabolism 88:3167-3176
398. Jeschke MG, Finnerty CC, Suman OE, Kulp G, Mlcak RP, Herndon DN (2007) The effect of oxandrolone on the endocrinologic, inflammatory, and hypermetabolic responses during the acute phase postburn. Annals of Surgery 246:351-360; discussion 360-352
399. Bulger EM, Jurkovich GJ, Farver CL, Klotz P, Maier RV (2004) Oxandrolone does not improve outcome of ventilator dependent surgical patients. Annals of Surgery 240:472-478; discussion 478-480
400. Harman SM, Metter EJ, Tobin JD, Pearson J, Blackman MR (2001) Longitudinal effects of aging on serum total and free testosterone levels in healthy men. Baltimore Longitudinal Study of Aging. Journal of Clinical Endocrinology and Metabolism 86:724-731.
401. Travison TG, Shackelton R, Araujo AB, Hall SA, Williams RE, Clark RV, O'Donnell AB, McKinlay JB (2008) The natural history of symptomatic androgen deficiency in men: onset, progression, and spontaneous remission. Journal of the American Geriatrics Society 56:831-839
402. Deslypere JP, Vermeulen A (1981) Aging and tissue androgens. Journal of Clinical Endocrinology and Metabolism 53:430-434
403. Deslypere JP, Vermeulen A (1985) Influence of age on steroid concentration in skin and striated muscle in women and in cardiac muscle and lung tissue in men. Journal of Clinical Endocrinology and Metabolism 60:648-653
404. Mohr BA, Guay AT, O'Donnell AB, McKinlay JB (2005) Normal, bound and nonbound testosterone levels in normally ageing men: results from the Massachusetts Male Ageing Study. Clinical Endocrinology 62:64-73
405. Corona G, Monami M, Rastrelli G, Aversa A, Tishova Y, Saad F, Lenzi A, Forti G, Mannucci E, Maggi M (2011) Testosterone and metabolic syndrome: a meta-analysis study. J Sex Med 8:272-283
406. Brand JS, van der Tweel I, Grobbee DE, Emmelot-Vonk MH, van der Schouw YT (2011) Testosterone, sex hormone-binding globulin and the metabolic syndrome: a systematic review and meta-analysis of observational studies. International Journal of Epidemiology 40:189-207
407. Ruige JB, Mahmoud AM, De Bacquer D, Kaufman JM (2011) Endogenous testosterone and cardiovascular disease in healthy men: a meta-analysis. Heart 97:870-875
408. Araujo AB, Dixon JM, Suarez EA, Murad MH, Guey LT, Wittert GA (2011) Clinical review: Endogenous testosterone and mortality in men: a systematic review and meta-analysis. Journal of Clinical Endocrinology and Metabolism 96:3007-3019
409. Toma M, McAlister FA, Coglianese EE, Vidi V, Vasaiwala S, Bakal JA, Armstrong PW, Ezekowitz JA (2012) Testosterone supplementation in heart failure: a meta-analysis. Circ Heart Fail 5:315-321
410. Borst SE, Shuster JJ, Zou B, Ye F, Jia H, Wokhlu A, Yarrow JF (2014) Cardiovascular risks and elevation of serum DHT vary by route of testosterone administration: a systematic review and meta-analysis. BMC Med 12:211
411. Onasanya O, Iyer G, Lucas E, Lin D, Singh S, Alexander GC (2016) Association between exogenous testosterone and cardiovascular events: an overview of systematic reviews. Lancet Diabetes Endocrinol
412. Alexander GC, Iyer G, Lucas E, Lin D, Singh S (2016) Cardiovascular Risks of Exogenous Testosterone Use Among Men: A Systematic Review and Meta-Analysis. American Journal of Medicine
413. Shores MM, Smith NL, Forsberg CW, Anawalt BD, Matsumoto AM (2012) Testosterone treatment and mortality in men with low testosterone levels. Journal of Clinical Endocrinology and Metabolism 97:2050-2058
414. Wu FC (2012) Caveat emptor: does testosterone treatment reduce mortality in men? Journal of Clinical Endocrinology and Metabolism 97:1884-1886
415. Gruenewald DA, Matsumoto AM (2003) Testosterone supplementation therapy for older men: potential benefits and risks. Journal of the American Geriatrics Society 51:101-115
416. Ly LP, Jimenez M, Zhuang TN, Celermajer DS, Conway AJ, Handelsman DJ (2001) A double-blind, placebo-controlled, randomized clinical trial of transdermal dihydrotestosterone gel on muscular strength, mobility, and quality of life in older men with partial androgen deficiency. Journal of Clinical Endocrinology and Metabolism 86:4078-4088.
417. Kunelius P, Lukkarinen O, Hannuksela ML, Itkonen O, Tapanainen JS (2002) The effects of transdermal dihydrotestosterone in the aging male: a prospective, randomized, double blind study. Journal of Clinical Endocrinology and Metabolism 87:1467-1472
418. Liu PY, Wishart SM, Handelsman DJ (2002) A double-blind, placebo-controlled, randomized clinical trial of recombinant human chorionic gonadotropin on muscle strength and physical function and activity in older men with partial age-related androgen deficiency. Journal of Clinical Endocrinology and Metabolism 87:3125-3135
419. Bhasin S, Calof OM, Storer TW, Lee ML, Mazer NA, Jasuja R, Montori VM, Gao W, Dalton JT (2006) Drug insight: Testosterone and selective androgen receptor modulators as anabolic therapies for chronic illness and aging. Nat Clin Pract Endocrinol Metab 2:146-159
420. Isidori AM, Giannetta E, Greco EA, Gianfrilli D, Bonifacio V, Isidori A, Lenzi A, Fabbri A (2005) Effects of testosterone on body composition, bone metabolism and serum lipid profile in middle-aged men: a meta-analysis. Clinical Endocrinology 63:280-293
421. Tracz MJ, Sideras K, Bolona ER, Haddad RM, Kennedy CC, Uraga MV, Caples SM, Erwin PJ, Montori VM (2006) Testosterone use in men and its effects on bone health. A systematic review and meta-analysis of randomized placebo-controlled trials. Journal of Clinical Endocrinology and Metabolism 91:2011-2016
422. Ottenbacher KJ, Ottenbacher ME, Ottenbacher AJ, Acha AA, Ostir GV (2006) Androgen treatment and muscle strength in elderly men: A meta-analysis. Journal of the American Geriatrics Society 54:1666-1673
423. Isidori AM, Giannetta E, Gianfrilli D, Greco EA, Bonifacio V, Aversa A, Isidori A, Fabbri A, Lenzi A (2005) Effects of testosterone on sexual function in men: results of a meta-analysis. Clinical Endocrinology 63:381-394
424. Bolona ER, Uraga MV, Haddad RM, Tracz MJ, Sideras K, Kennedy CC, Caples SM, Erwin PJ, Montori VM (2007) Testosterone use in men with sexual dysfunction: a systematic review and meta-analysis of randomized placebo-controlled trials. Mayo Clinic Proceedings 82:20-28
425. Calof OM, Singh AB, Lee ML, Kenny AM, Urban RJ, Tenover JL, Bhasin S (2005) Adverse events associated with testosterone replacement in middle-aged and older men: a meta-analysis of randomized, placebo-controlled trials. Journals of Gerontology Series A, Biological Sciences and Medical Sciences 60:1451-1457
426. Liverman CT, Blazer DG eds. 2004 Testosterone and Aging: Clinical Research Directions. Washington, DC: Institute of Medicine: The National Academies Press
427. Snyder PJ, Bhasin S, Cunningham GR, Matsumoto AM, Stephens-Shields AJ, Cauley JA, Gill TM, Barrett-Connor E, Swerdloff RS, Wang C, Ensrud KE, Lewis CE, Farrar JT, Cella D, Rosen RC, Pahor M, Crandall JP, Molitch ME, Cifelli D, Dougar D, Fluharty L, Resnick SM, Storer TW, Anton S, Basaria S, Diem SJ, Hou X, Mohler ER, 3rd, Parsons JK, Wenger NK, Zeldow B, Landis JR, Ellenberg SS, Testosterone Trials I (2016) Effects of Testosterone Treatment in Older Men. New England Journal of Medicine 374:611-624
428. Orwoll ES (2016) Establishing a Framework--Does Testosterone Supplementation Help Older Men? New England Journal of Medicine 374:682-683
429. Rossouw JE, Anderson GL, Prentice RL, LaCroix AZ, Kooperberg C, Stefanick ML, Jackson RD, Beresford SA, Howard BV, Johnson KC, Kotchen JM, Ockene J (2002) Risks and benefits of estrogen plus progestin in healthy postmenopausal women: principal results From the Women's Health Initiative randomized controlled trial. Jama 288:321-333
430. Kalin MF, Zumoff B (1990) Sex hormones and coronary disease: a review of the clinical studies. Steroids 55:330-352
431. Zhang Y, Ouyang P, Post WS, Dalal D, Vaidya D, Blasco-Colmenares E, Soliman EZ, Tomaselli GF, Guallar E (2011) Sex-steroid hormones and electrocardiographic QT-interval duration: findings from the third National Health and Nutrition Examination Survey and the Multi-Ethnic Study of Atherosclerosis. American Journal of Epidemiology 174:403-411
432. Carnes CA, Dech SJ (2002) Effects of dihydrotestosterone on cardiac inward rectifier K(+) current. International Journal of Andrology 25:210-214
433. Liu XK, Katchman A, Whitfield BH, Wan G, Janowski EM, Woosley RL, Ebert SN (2003) In vivo androgen treatment shortens the QT interval and increases the densities of inward and delayed rectifier potassium currents in orchiectomized male rabbits. Cardiovascular Research 57:28-36
434. Fulop L, Banyasz T, Szabo G, Toth IB, Biro T, Lorincz I, Balogh A, Peto K, Miko I, Nanasi PP (2006) Effects of sex hormones on ECG parameters and expression of cardiac ion channels in dogs. Acta Physiol (Oxf) 188:163-171
435. Ridley JM, Shuba YM, James AF, Hancox JC (2008) Modulation by testosterone of an endogenous hERG potassium channel current. Journal of Physiology and Pharmacology 59:395-407
436. Wu ZY, Chen K, Haendler B, McDonald TV, Bian JS (2008) Stimulation of N-terminal truncated isoform of androgen receptor stabilizes human ether-a-go-go-related gene-encoded potassium channel protein via activation of extracellular signal regulated kinase 1/2. Endocrinology 149:5061-5069
437. Charbit B, Christin-Maitre S, Demolis JL, Soustre E, Young J, Funck-Brentano C (2009) Effects of testosterone on ventricular repolarization in hypogonadic men. American Journal of Cardiology 103:887-890
438. Pecori Giraldi F, Toja PM, Filippini B, Michailidis J, Scacchi M, Stramba Badiale M, Cavagnini F (2010) Increased prevalence of prolonged QT interval in males with primary or secondary hypogonadism: a pilot study. International Journal of Andrology 33:e132-138
439. van Noord C, Rodenburg EM, Stricker BH (2011) Invited commentary: sex-steroid hormones and QT-interval duration. American Journal of Epidemiology 174:412-415
440. Sieveking DP, Lim P, Chow RW, Dunn LL, Bao S, McGrath KC, Heather AK, Handelsman DJ, Celermajer DS, Ng MK (2010) A sex-specific role for androgens in angiogenesis. Journal of Experimental Medicine 207:345-352
441. Lecce L, Lam YT, Lindsay LA, Yuen SC, Simpson PJ, Handelsman DJ, Ng MK (2014) Aging impairs VEGF-mediated, androgen-dependent regulation of angiogenesis. Molecular Endocrinology 28:1487-1501
442. Wu FC, von Eckardstein A (2003) Androgens and coronary artery disease. Endocrine Reviews 24:183-217
443. Khaw KT, Dowsett M, Folkerd E, Bingham S, Wareham N, Luben R, Welch A, Day N (2007) Endogenous testosterone and mortality due to all causes, cardiovascular disease, and cancer in men: European prospective investigation into cancer in Norfolk (EPIC-Norfolk) Prospective Population Study. Circulation 116:2694-2701
444. Laughlin GA, Barrett-Connor E, Bergstrom J (2008) Low serum testosterone and mortality in older men. Journal of Clinical Endocrinology and Metabolism 93:68-75
445. Smith GD, Ben-Shlomo Y, Beswick A, Yarnell J, Lightman S, Elwood P (2005) Cortisol, testosterone, and coronary heart disease: prospective evidence from the Caerphilly study. Circulation 112:332-340
446. Araujo AB, Kupelian V, Page ST, Handelsman DJ, Bremner WJ, McKinlay JB (2007) Sex steroids and all-cause and cause-specific mortality in men. Archives of Internal Medicine 167:1252-1260
447. Maggio M, Lauretani F, Ceda GP, Bandinelli S, Ling SM, Metter EJ, Artoni A, Carassale L, Cazzato A, Ceresini G, Guralnik JM, Basaria S, Valenti G, Ferrucci L (2007) Relationship between low levels of anabolic hormones and 6-year mortality in older men: the aging in the Chianti Area (InCHIANTI) study. Archives of Internal Medicine 167:2249-2254
448. Xu L, Freeman G, Cowling BJ, Schooling CM (2013) Testosterone therapy and cardiovascular events among men: a systematic review and meta-analysis of placebo-controlled randomized trials. BMC Med 11:108
449. Handelsman DJ (2011) An old emperor finds new clothing: rejuvenation in our time. Asian Journal of Andrology 13:125-129
450. Haring R, Teumer A, Volker U, Dorr M, Nauck M, Biffar R, Volzke H, Baumeister SE, Wallaschofski H (2013) Mendelian randomization suggests non-causal associations of testosterone with cardiometabolic risk factors and mortality. Andrology 1:17-23
451. Zhao J, Jiang C, Lam TH, Liu B, Cheng KK, Xu L, Au Yeung SL, Zhang W, Leung GM, Schooling CM (2014) Genetically predicted testosterone and cardiovascular risk factors in men: a Mendelian randomization analysis in the Guangzhou Biobank Cohort Study. International Journal of Epidemiology 43:140-148
452. Gong J, Zubair N (2015) Commentary: Mendelian randomization, testosterone, and cardiovascular disease. International Journal of Epidemiology 44:621-622
453. Swerdlow AJ, Higgins CD, Schoemaker MJ, Wright AF, Jacobs PA (2005) Mortality in patients with Klinefelter syndrome in Britain: a cohort study. Journal of Clinical Endocrinology and Metabolism 90:6516-6522
454. Roddam AW, Allen NE, Appleby P, Key TJ (2008) Endogenous sex hormones and prostate cancer: a collaborative analysis of 18 prospective studies. Journal of the National Cancer Institute 100:170-183
455. Boyle P, Koechlin A, Bota M, d'Onofrio A, Zaridze DG, Perrin P, Fitzpatrick J, Burnett AL, Boniol M (2016) Endogenous and exogenous testosterone and the risk of prostate cancer and increased prostate specific antigen (PSA): a meta-analysis. BJU International
456. Millar AC, Lau AN, Tomlinson G, Kraguljac A, Simel DL, Detsky AS, Lipscombe LL (2016) Predicting low testosterone in aging men: a systematic review. Cmaj 188:E321-330
457. Huo S, Scialli AR, McGarvey S, Hill E, Tugertimur B, Hogenmiller A, Hirsch AI, Fugh-Berman A (2016) Treatment of Men for "Low Testosterone": A Systematic Review. PLoS One 11:e0162480
458. Conway AJ, Handelsman DJ, Lording DW, Stuckey B, Zajac JD (2000) Use, misuse and abuse of androgens: The Endocrine Society of Australia consensus guidelines for androgen prescribing. Medical Journal of Australia 172:220-224
459. Petak SM, Nankin HR, Spark RF, Swerdloff RS, Rodriguez-Rigau LJ, American Association of Clinical E (2002) American Association of Clinical Endocrinologists Medical Guidelines for clinical practice for the evaluation and treatment of hypogonadism in adult male patients--2002 update. Endocr Pract 8:440-456
460. Nieschlag E, Swerdloff R, Behre HM, Gooren LJ, Kaufman JM, Legros JJ, Lunenfeld B, Morley JE, Schulman C, Wang C, Weidner W, Wu FC (2005) Investigation, treatment and monitoring of late-onset hypogonadism in males: ISA, ISSAM, and EAU recommendations. International Journal of Andrology 28:125-127
461. Bhasin S, Cunningham GR, Hayes FJ, Matsumoto AM, Snyder PJ, Swerdloff RS, Montori VM (2006) Testosterone therapy in adult men with androgen deficiency syndromes: an endocrine society clinical practice guideline. Journal of Clinical Endocrinology and Metabolism 91:1995-2010
462. Handelsman DJ (2012) Pharmacoepidemiology of testosterone prescribing in Australia, 1992-2010. Medical Journal of Australia 196:642-645
463. Gan EH, Pattman S, S HSP, Quinton R (2013) A UK epidemic of testosterone prescribing, 2001-2010. Clinical Endocrinology 79:564-570
464. Nigro N, Christ-Crain M (2012) Testosterone treatment in the aging male: myth or reality? Swiss Medical Weekly 142:w13539
465. Bhasin S, Singh AB, Mac RP, Carter B, Lee MI, Cunningham GR (2003) Managing the risks of prostate disease during testosterone replacement therapy in older men: recommendations for a standardized monitoring plan. Journal of Andrology 24:299-311
466. Tan RS, Salazar JA (2004) Risks of testosterone replacement therapy in ageing men. Expert Opin Drug Saf 3:599-606
467. Baillargeon J, Urban RJ, Ottenbacher KJ, Pierson KS, Goodwin JS (2013) Trends in androgen prescribing in the United States, 2001 to 2011. JAMA Intern Med 173:1465-1466
468. Handelsman DJ (2013) Global trends in testosterone prescribing, 2000-2011: expanding the spectrum of prescription drug misuse. Medical Journal of Australia 199:548-551
469. Yeap BB, Grossmann M, McLachlan RI, Handelsman DJ, Wittert GA, Conway AJ, Stuckey BG, Lording DW, Allan CA, Zajac JD, Burger HG (2016) Endocrine Society of Australia position statement on male hypogonadism (part 1): assessment and indications for testosterone therapy. Medical Journal of Australia 205:173-178
470. Yeap BB, Grossmann M, McLachlan RI, Handelsman DJ, Wittert GA, Conway AJ, Stuckey BG, Lording DW, Allan CA, Zajac JD, Burger HG (2016) Endocrine Society of Australia position statement on male hypogonadism (part 2): treatment and therapeutic considerations. Medical Journal of Australia 205:228-231
471. Vandekerckhove P, Lilford R, Vail A, Hughes E (2000) Androgens versus placebo or no treatment for idiopathic oligo/asthenospermia. Cochrane Database Syst Rev:CD000150
472. Gabrielsen JS, Najari BB, Alukal JP, Eisenberg ML (2016) Trends in Testosterone Prescription and Public Health Concerns. Urologic Clinics of North America 43:261-271
473. Handelsman DJ (2004) Trends and regional differences in testosterone prescribing in Australia, 1991-2001. Medical Journal of Australia 181:419-422
474. Layton JB, Li D, Meier CR, Sharpless J, Sturmer T, Jick SS, Brookhart MA (2014) Testosterone Lab Testing and Initiation in the United Kingdom and the United States, 2000-2011. Journal of Clinical Endocrinology and Metabolism:jc20133570
475. Rao PK, Boulet SL, Mehta A, Hotaling J, Eisenberg ML, Honig SC, Warner L, Kissin DM, Nangia AK, Ross LS (2016) Trends in Testosterone Replacement Therapy Use Among Reproductive-Age US Men, 2003-2013. Journal of Urology
476. Jasuja GK, Bhasin S, Reisman JI, Berlowitz DR, Rose AJ (2015) Ascertainment of Testosterone Prescribing Practices in the VA. Medical Care 53:746-752
477. Bhasin S, Cunningham GR, Hayes FJ, Matsumoto AM, Snyder PJ, Swerdloff RS, Montori VM (2010) Testosterone therapy in men with androgen deficiency syndromes: an Endocrine Society clinical practice guideline. Journal of Clinical Endocrinology and Metabolism 95:2536-2559
478. Wang C, Nieschlag E, Swerdloff R, Behre HM, Hellstrom WJ, Gooren LJ, Kaufman JM, Legros JJ, Lunenfeld B, Morales A, Morley JE, Schulman C, Thompson IM, Weidner W, Wu FC (2009) Investigation, treatment, and monitoring of late-onset hypogonadism in males: ISA, ISSAM, EAU, EAA, and ASA recommendations. Journal of Andrology 30:1-9
479. Handelsman DJ 2010 Androgen Physiology, Pharmacology and Abuse. In: DeGroot LJ, Jameson JL eds. Endocrinology. 6th ed. Philadelphia: Elsevier Saunders; 2469-2498
480. Herlihy AS, Halliday JL, Cock ML, McLachlan RI (2011) The prevalence and diagnosis rates of Klinefelter syndrome: an Australian comparison. Medical Journal of Australia 194:24-28
481. Mulligan T, Frick MF, Zuraw QC, Stemhagen A, McWhirter C (2006) Prevalence of hypogonadism in males aged at least 45 years: the HIM study. International Journal of Clinical Practice 60:762-769
482. Araujo AB, O'Donnell AB, Brambilla DJ, Simpson WB, Longcope C, Matsumoto AM, McKinlay JB (2004) Prevalence and incidence of androgen deficiency in middle-aged and older men: estimates from the Massachusetts Male Aging Study. Journal of Clinical Endocrinology and Metabolism 89:5920-5926
483. Haring R, Ittermann T, Volzke H, Krebs A, Zygmunt M, Felix SB, Grabe HJ, Nauck M, Wallaschofski H (2010) Prevalence, incidence and risk factors of testosterone deficiency in a population-based cohort of men: results from the study of health in Pomerania. Aging Male 13:247-257
484. Wu FC, Tajar A, Beynon JM, Pye SR, Silman AJ, Finn JD, O'Neill TW, Bartfai G, Casanueva FF, Forti G, Giwercman A, Han TS, Kula K, Lean ME, Pendleton N, Punab M, Boonen S, Vanderschueren D, Labrie F, Huhtaniemi IT (2010) Identification of late-onset hypogonadism in middle-aged and elderly men. New England Journal of Medicine 363:123-135
485. Hoberman JM, Yesalis CE (1995) The history of synthetic testosterone. Scientific American 272:76-81
486. Franke WW, Berendonk B (1997) Hormonal doping and androgenization of athletes: a secret program of the German Democratic Republic government. Clinical Chemistry 43:1262-1279
487. Bhasin S, Woodhouse L, Casaburi R, Singh AB, Bhasin D, Berman N, Chen X, Yarasheski KE, Magliano L, Dzekov C, Dzekov J, Bross R, Phillips J, Sinha-Hikim I, Shen R, Storer TW (2001) Testosterone dose-response relationships in healthy young men. Am J Physiol Endocrinol Metab 281:E1172-1181
488. Pope HG, Jr., Kouri EM, Hudson JI (2000) Effects of supraphysiologic doses of testosterone on mood and aggression in normal men: a randomized controlled trial. Archives of General Psychiatry 57:133-140; discussion 155-136
489. Kanayama G, Hudson JI, Pope HG, Jr. (2008) Long-term psychiatric and medical consequences of anabolic-androgenic steroid abuse: A looming public health concern? Drug and Alcohol Dependence 98:1-12
490. Sagoe D, Molde H, Andreassen CS, Torsheim T, Pallesen S (2014) The global epidemiology of anabolic-androgenic steroid use: a meta-analysis and meta-regression analysis. Annals of Epidemiology 24:383-398
491. Buckley WE, Yesalis CE, Freidl KE, Anderson WA, Streit AL, Wright JE (1988) Estimated prevalence of anabolic steroid use among male high school students. Journal of the American Medical Association 260:3441-3445
492. Nilsson S (1995) Androgenic anabolic steroid use among male adolescents in Falkenberg. Europen Journal of Clinical Pharmacology 48:9-11
493. Handelsman DJ, Gupta L (1997) Prevalence and risk factors for anabolic-androgenic steroid abuse in Australian secondary school students. International Journal of Andrology 20:159-164
494. Lambert MI, Titlestad SD, Schwellnus MP (1998) Prevalence of androgenic-anabolic steroid use in adolescents in two regions of South Africa. South African Medical Journal 88:876-880
495. Ferenchick GS (1996) Validity of self-report in identifying anabolic steroid use among weightlifters. Journal of General Internal Medicine 11:554-556
496. Pope HG, Kouri EM, Powell KF, Campbell C, Katz DL (1996) Anabolic-androgenic steroid use among 133 prisoners. Comprehensive Psychiatry 37:322-327
497. Isacsson G, Garle M, Ljung EB, Asgard U, Bergmen U (1998) Anabolic steroids and violent crime - an epidemiological study at a jail in Stockholm, Sweden. Comprehensive Psychiatry 39:203-205
498. Catlin DH, Ahrens BD, Kucherova Y (2002) Detection of norbolethone, an anabolic steroid never marketed, in athletes' urine. Rapid Communication in Mass Spectrometry 16:1273-1275
499. Death AK, McGrath KC, Kazlauskas R, Handelsman DJ (2004) Tetrahydrogestrinone is a potent androgen and progestin. Journal of Clinical Endocrinology and Metabolism 89:2498-2500
500. Catlin DH, Sekera MH, Ahrens BD, Starcevic B, Chang YC, Hatton CK (2004) Tetrahydrogestrinone: discovery, synthesis, and detection. Rapid Communication in Mass Spectrometry 18:1245-1249
501. Sekera MH, Ahrens BD, Chang YC, Starcevic B, Georgakopoulos C, Catlin DH (2005) Another designer steroid: discovery, synthesis, and detection of 'madol' in urine. Rapid Communication in Mass Spectrometry 19:781-784
502. Handelsman DJ, Heather A (2008) Androgen abuse in sports. Asian Journal of Andrology 10:403-415
503. vandenBerg P, Neumark-Sztainer D, Cafri G, Wall M (2007) Steroid use among adolescents: longitudinal findings from Project EAT. Pediatrics 119:476-486
504. McCabe SE, Brower KJ, West BT, Nelson TF, Wechsler H (2007) Trends in non-medical use of anabolic steroids by U.S. college students: results from four national surveys. Drug and Alcohol Dependence 90:243-251
505. Holma PK (1977) Effects of an anabolic steroid (metandienone) on spermatogenesis. Contraception 15:151-162
506. Knuth UA, Maniera H, Nieschlag E (1989) Anabolic steroids and semen parameters in bodybuilders. Fertility and Sterility 52:1041-1047
507. Turek PJ, Williams RH, Gilbaugh JH, Lipshultz LI (1995) The reversibility of anabolic steroid-induced azoospermia. Journal of Urology 153:1628-1630
508. Sorensen M, Ingerslev HJ (1995) Azoospermia in two bodybuilders taking anabolic steroids. Ugeskrift for Laeger 157:1044-1045
509. Gazvani MR, Buckett W, Luckas MJ, Aird IA, Hipkin LJ, Lewis-Jones DI (1997) Conservative management of azoospermia following steroid abuse. Human Reproduction 12:1706-1708
510. Reyes RJ, Zicchi S, Hamed H, Chaudary MA, Fentiman IS (1995) Surgical correction of gynaecomastia in bodybuilders. British Journal of Clinical Practice 49:177-179
511. Friedl KE (1990) Reappraisal of health risks associated with use of high doses of oral and injectable androgenic steroids. NIDA Research Monograph 102:142-177
512. Sklarek HM, Mantovani RP, Erens E, Heisler D, Niederman MS, Fein AM (1984) AIDS in a bodybuilder using anabolic steroids [letter]. New England Journal of Medicine 311:1701
513. Nemechek PM (1991) Anabolic steroid users--another potential risk group for HIV infection [letter]. New England Journal of Medicine 325:357
514. Henrion R, Mandelbrot L, Delfieu D (1992) HIV contamination after injections of anabolic steroids (letter). Presse Medicale 21:218
515. Rich JD, Dickinson BP, Merriman NA, Flanigan TP (1998) Hepatitis C virus infection related to anabolic-androgenic steroid injection in a recreational weight lifter [letter]. American Journal of Gastroenterology 93:1598
516. Aitken C, Delalande C, Stanton K (2002) Pumping iron, risking infection? Exposure to hepatitis C, hepatitis B and HIV among anabolic-androgenic steroid injectors in Victoria, Australia. Drug and Alcohol Dependence 65:303-308
517. Rich JD, Dickinson BP, Feller A, Pugatch D, Mylonakis E (1999) The infectious complications of anabolic-androgenic steroid injection. International Journal of Sports Medicine 20:563-566
518. Khankhanian NK, Hammers YA (1992) Exuberant local tissue reaction to intramuscular injection of nandrolone decanoate (Deca-Durabolin) - a steroid compound in sesame seed oil base - mimicking soft tissue malignant tumors: a case report and review of the literature. Military Medicine 157:670-674
519. Evans NA (1997) Local complications of self administered anabolic steroid injections. British Journal of Sports Medicine 31:349-350
520. Freeman BJ, Rooker GD (1995) Spontaneous rupture of the anterior cruciate ligament after anabolic steroids. British Journal of Sports Medicine 29:274-275
521. Daniels JM, van Westerloo DJ, de Hon OM, Frissen PH (2006) [Rhabdomyolysis in a bodybuilder using steroids]. Nederlands Tijdschrift Voor Geneeskunde 150:1077-1080
522. Lepori M, Perren A, Gallino A (2002) The popliteal-artery entrapment syndrome in a patient using anabolic steroids. New England Journal of Medicine 346:1254-1255
523. Sahraian MA, Mottamedi M, Azimi AR, Moghimi B (2004) Androgen-induced cerebral venous sinus thrombosis in a young body builder: case report. BMC Neurol 4:22
524. Liljeqvist S, Hellden A, Bergman U, Soderberg M (2008) Pulmonary embolism associated with the use of anabolic steroids. Eur J Intern Med 19:214-215
525. Alaraj AM, Chamoun RB, Dahdaleh NS, Haddad GF, Comair YG (2005) Spontaneous subdural haematoma in anabolic steroids dependent weight lifters: reports of two cases and review of literature. Acta Neurochirurgica 147:85-87; discussion 87-88
526. Petersson A, Garle M, Granath F, Thiblin I (2007) Convulsions in users of anabolic androgenic steroids: possible explanations. Journal of Clinical Psychopharmacology 27:723-725
527. Pope HG, Katz DL (1988) Affective and psychotic symptoms associated with anabolic steroid use. American Journal of Psychiatry 145:487-490
528. Bahrke MS, Yesalis CE, Wright JE (1996) Psychological and behavioural effects of endogenous testosterone levels and anabolic-androgenic steroids among male. An update. Sports Medicine 22:367-390
529. Rockhold RW (1993) Cardiovascular toxicity of anabolic steroids. Annual Review of Pharmacology and Toxicology 33:497-520
530. Melchert RB, Welder AA (1995) Cardiovascular effects of androgenic-anabolic steroids. Medicine and Science in Sports and Exercise 27:1252-1262
531. Sullivan ML, Martinez CM, Gennis P, Gallagher EJ (1998) The cardiac toxicity of anabolic steroids. Progress in Cardiovascular Diseases 41:1-15
532. Dhar R, Stout CW, Link MS, Homoud MK, Weinstock J, Estes NA, 3rd (2005) Cardiovascular toxicities of performance-enhancing substances in sports. Mayo Clinic Proceedings 80:1307-1315
533. Furlanello F, Serdoz LV, Cappato R, De Ambroggi L (2007) Illicit drugs and cardiac arrhythmias in athletes. Eur J Cardiovasc Prev Rehabil 14:487-494
534. Larkin GL (1991) Carcinoma of the prostate. New England Journal of Medicine 324:1892
535. Roberts JT, Essenhigh DM (1986) Adenocarcinoma of prostate in 40 year old body-builder. Lancet 2:742
536. Nakata S, Hasumi M, Sato J, Ogawa A, Yamanaka H (1997) Prostate cancer associated with long-term intake of patent medicine containing methyltestosterone: a case report. Hinyokika Kiyo - Acta Urologica Japonica 43:791-793
537. Hartgens F, Cheriex EC, Kuipers H (2003) Prospective echocardiographic assessment of androgenic-anabolic steroids effects on cardiac structure and function in strength athletes. International Journal of Sports Medicine 24:344-351
538. Chung T, Kelleher S, Liu PY, Conway AJ, Kritharides L, Handelsman DJ (2007) Effects of testosterone and nandrolone on cardiac function: a randomized, placebo-controlled study. Clinical Endocrinology 66:235-245
539. Jin B, Turner L, Walters WAW, Handelsman DJ (1996) Androgen or estrogen effects on the human prostate. Journal of Clinical Endocrinology and Metabolism 81:4290-4295
540. Palatini P, Giada F, Garavelli G, Sinisi F, Mario L, Michieletto M, Baldo-Enzi G (1996) Cardiovascular effects of anabolic steroids in weight-trained subjects. Journal of Clinical Pharmacology and New Drugs 36:1132-1140
541. Krieg A, Scharhag J, Albers T, Kindermann W, Urhausen A (2007) Cardiac tissue Doppler in steroid users. International Journal of Sports Medicine 28:638-643
542. D'Andrea A, Caso P, Salerno G, Scarafile R, De Corato G, Mita C, Di Salvo G, Severino S, Cuomo S, Liccardo B, Esposito N, Calabro R (2007) Left ventricular early myocardial dysfunction after chronic misuse of anabolic androgenic steroids: a Doppler myocardial and strain imaging analysis. British Journal of Sports Medicine 41:149-155
543. Lane HA, Grace F, Smith JC, Morris K, Cockcroft J, Scanlon MF, Davies JS (2006) Impaired vasoreactivity in bodybuilders using androgenic anabolic steroids. European Journal of Clinical Investigation 36:483-488
544. Nottin S, Nguyen LD, Terbah M, Obert P (2006) Cardiovascular effects of androgenic anabolic steroids in male bodybuilders determined by tissue Doppler imaging. American Journal of Cardiology 97:912-915
545. Urhausen A, Albers T, Kindermann W (2004) Are the cardiac effects of anabolic steroid abuse in strength athletes reversible? Heart 90:496-501
546. Climstein M, O'Shea P, Adams KJ, DeBeliso M (2003) The effects of anabolic-androgenic steroids upon resting and peak exercise left ventricular heart wall motion kinetics in male strength and power athletes. Journal of Science and Medicine in Sport 6:387-397
547. Grace F, Sculthorpe N, Baker J, Davies B (2003) Blood pressure and rate pressure product response in males using high-dose anabolic androgenic steroids (AAS). Journal of Science and Medicine in Sport 6:307-312
548. Karila TA, Karjalainen JE, Mantysaari MJ, Viitasalo MT, Seppala TA (2003) Anabolic androgenic steroids produce dose-dependant increase in left ventricular mass in power atheletes, and this effect is potentiated by concomitant use of growth hormone. International Journal of Sports Medicine 24:337-343
549. Sader MA, Griffiths KA, McCredie RJ, Handelsman DJ, Celermajer DS (2001) Androgenic anabolic steroids and arterial structure and function in male bodybuilders. Journal of the American College of Cardiology 37:224-230
550. Di Bello V, Giorgi D, Bianchi M, Bertini A, Caputo MT, Valenti G, Furioso O, Alessandri L, Paterni M, Giusti C (1999) Effects of anabolic-androgenic steroids on weight-lifters' myocardium: an ultrasonic videodensitometric study. Medicine and Science in Sports and Exercise 31:514-521
551. Dickerman RD, Schaller F, Zachariah NY, McConathy WJ (1997) Left ventricular size and function in elite bodybuilders using anabolic steroids. Clinical Journal of Sports Medicine 7:90-93
552. Di Bello V, Pedrinelli R, Giorgi D, Bertini A, Talarico L, Caputo MT, Massimiliano B, Dell'Omo G, Paterni M, Giusti C (1997) Ultrasonic videodensitometric analysis of two different models of left ventricular hypertrophy. Athlete's heart and hypertension. Hypertension 29:937-944
553. Thiblin I, Petersson A (2005) Pharmacoepidemiology of anabolic androgenic steroids: a review. Fundamental and Clinical Pharmacology 19:27-44
554. Sarna S, Sahi T, Koskenvuo M, Kaprio J (1993) Increased life expectancy of world class male athletes. Medicine and Science in Sports and Exercise 25:237-244
555. Sarna S, Kaprio J, Kujala UM, Koskenvuo M (1997) Health status of former elite athletes. The Finnish experience. Aging 9:35-41
556. Parssinen M, Kujala U, Vartiainen E, Sarna S, Seppala T (2000) Increased premature mortality of competitive powerlifters suspected to have used anabolic agents. International Journal of Sports Medicine 21:225-227
557. Kashkin KB, Kleber HD (1989) Hooked on hormones? An anabolic steroid addiction hypothesis. Journal of the American Medical Association 262:3166-3170
558. Fingerhood MI, Sullivan JT, Testa M, Jasinski DR (1997) Abuse liability of testosterone. Journal of Psychopharmacology 11:59-63
559. Gazvani MR, Buckett W, Luckas MJ, Aird IA, Hipkin LJ, Lewis-Jones DI (1997) Conservative management of azoospermia following steroid abuse. Hum Reprod 12:1706-1708
560. Gill GV (1998) Anabolic steroid induced hypogonadism treated with human chorionic gonadotropin. Postgrad Med J 74:45-46
561. Boyadjiev NP, Georgieva KN, Massaldjieva RI, Gueorguiev SI (2000) Reversible hypogonadism and azoospermia as a result of anabolic-androgenic steroid use in a bodybuilder with personality disorder. A case report. J Sports Med Phys Fitness 40:271-274
562. Torres-Calleja J, Gonzalez-Unzaga M, DeCelis-Carrillo R, Calzada-Sanchez L, Pedron N (2001) Effect of androgenic anabolic steroids on sperm quality and serum hormone levels in adult male bodybuilders. Life Sci 68:1769-1774
563. Shankara-Narayana N, Yu C, Savkovic S, Desai R, Fennell C, Turner L, Jayadev V, Conway AJ, Kockx M, Ridley L, Kritharides L, Handelsman DJ (2020) Rate and Extent of Recovery from Reproductive and Cardiac Dysfunction Due to Androgen Abuse in Men. J Clin Endocrinol Metab 105
564. Menon DK (2003) Successful treatment of anabolic steroid-induced azoospermia with human chorionic gonadotropin and human menopausal gonadotropin. Fertility and Sterility 79 Suppl 3:1659-1661
565. Drakeley A, Gazvani R, Lewis-Jones I (2004) Duration of azoospermia following anabolic steroids. Fertility and Sterility 81:226
566. Nejat RJ, Rashid HH, Bagiella E, Katz AE, Benson MC (2000) A prospective analysis of time to normalization of serum testosterone after withdrawal of androgen deprivation therapy. Journal of Urology 164:1891-1894
567. Kaku H, Saika T, Tsushima T, Ebara S, Senoh T, Yamato T, Nasu Y, Kumon H (2006) Time course of serum testosterone and luteinizing hormone levels after cessation of long-term luteinizing hormone-releasing hormone agonist treatment in patients with prostate cancer. Prostate 66:439-444
568. Goldberg L, MacKinnon DP, Elliot DL, Moe EL, Clarke G, Cheong J (2000) The adolescents training and learning to avoid steroids program: preventing drug use and promoting health behaviors. Archives of Pediatrics and Adolescent Medicine 154:332-338
569. Behre HM, Kliesch S, Leifke E, Link TM, Nieschlag E (1997) Long-term effect of testosterone therapy on bone mineral density in hypogonadal men. Journal of Clinical Endocrinology and Metabolism
82:2386-2390
570. Jockenhovel F, Vogel E, Reinhardt W, Reinwein D (1997) Effects of various modes of androgen substitution therapy on erythropoiesis. European Journal of Medical Research 2:293-298
571. Wang C, Swedloff RS, Iranmanesh A, Dobs A, Snyder PJ, Cunningham G, Matsumoto AM, Weber T, Berman N (2000) Transdermal testosterone gel improves sexual function, mood, muscle strength, and body composition parameters in hypogonadal men. Testosterone Gel Study Group. Journal of Clinical Endocrinology and Metabolism 85:2839-2853
572. Aminorroaya A, Kelleher S, Conway AJ, Ly LP, Handelsman DJ (2005) Adequacy of androgen replacement influences bone density response to testosterone in androgen-deficient men. European Journal of Endocrinology 152:881-886
573. Wong FH, Pun KK, Wang C (1993) Loss of bone mass in patients with Klinefelter's syndrome despite sufficient testosterone replacement. Osteoporosis International 3:3-7
574. Ishizaka K, Suzuki M, Kageyama Y, Kihara K, Yoshida K (2002) Bone mineral density in hypogonadal men remains low after long-term testosterone replacement. Asian Journal of Andrology 4:117-121
575. Anderson RA, Wallace AM, Sattar N, Kumar N, Sundaram K (2003) Evidence for tissue selectivity of the synthetic androgen 7 alpha-methyl-19-nortestosterone in hypogonadal men. Journal of Clinical Endocrinology and Metabolism 88:2784-2793
576. Zacharin MR, Pua J, Kanumakala S (2003) Bone mineral density outcomes following long-term treatment with subcutaneous testosterone pellet implants in male hypogonadism. Clinical Endocrinology 58:691-695
577. Schubert M, Bullmann C, Minnemann T, Reiners C, Krone W, Jockenhovel F (2003) Osteoporosis in male hypogonadism: responses to androgen substitution differ among men with primary and secondary hypogonadism. Hormone Research 60:21-28
578. Finkelstein JS, Klibanski A, Neer RM (1996) A longitudinal evaluation of bone mineral density in adult men with histories of delayed puberty. Journal of Clinical Endocrinology and Metabolism 81:1152-1155
579. Finkelstein JS, Neer RM, Biller BM, Crawford JD, Klibanski A (1992) Osteopenia in men with a history of delayed puberty. New England Journal of Medicine 326:600-604
580. Huhtaniemi IT, Pye SR, Limer KL, Thomson W, O'Neill TW, Platt H, Payne D, John SL, Jiang M, Boonen S, Borghs H, Vanderschueren D, Adams JE, Ward KA, Bartfai G, Casanueva F, Finn JD, Forti G, Giwercman A, Han TS, Kula K, Lean ME, Pendleton N, Punab M, Silman AJ, Wu FC (2009) Increased estrogen rather than decreased androgen action is associated with longer androgen receptor CAG repeats. Journal of Clinical Endocrinology and Metabolism 94:277-284
581. Kicman AT (2008) Pharmacology of anabolic steroids. British Journal of Pharmacology 154:502-521
582. Dalton JT, Mukherjee A, Zhu Z, Kirkovsky L, Miller DD (1998) Discovery of nonsteroidal androgens. Biochemical and Biophysical Research Communications 244:1-4
583. Thevis M, Schanzer W (2008) Mass spectrometry of selective androgen receptor modulators. Journal of Mass Spectrometry 43:865-876
584. Lubahn D, Joseph DR, Sullivan PM, Williard HF, French FS, Wilson EM (1988) Cloning of the human androgen receptor complementary DNA and localisation to the X-chromosome. Science 240:327-330
585. Chang CS, Kokontis J, Liao ST (1988) Molecular cloning of human and rat complementary DNA encoding androgen receptors. Science 240:324-326
586. Trapman J, Klaassen P, Kuiper GG, van der Korput JA, Faber PW, van Rooij HC, Geurts van Kessel A, Voorhorst MM, Mulder E, Brinkmann AO (1988) Cloning, structure and expression of a cDNA encoding the human androgen receptor. Biochemical and Biophysical Research Communications 153:241-248
587. Wilson JD (1980) The use and misuse of androgens. Metabolism: Clinical and Experimental 29:1278-1295
588. Negro-Vilar A (1999) Selective androgen receptor modulators (SARMs): a novel approach to androgen therapy for the new millennium. Journal of Clinical Endocrinology and Metabolism 84:3459-3462
589. Bhasin S, Jasuja R (2009) Selective androgen receptor modulators as function promoting therapies. Current Opinion in Clinical Nutrition and Metabolic Care 12:232-240
590. Handelsman DJ, Conway AJ, Boylan LM (1990) Pharmacokinetics and pharmacodynamics of testosterone pellets in man. Journal of Clinical Endocrinology and Metabolism 71:216-222
591. Deansley R, Parkes AS (1938) Further experiments on the administration of hormones by the subcutaneous implantation of tablets. Lancet ii:606-608
592. Kelleher S, Howe C, Conway AJ, Handelsman DJ (2004) Testosterone release rate and duration of action of testosterone pellet implants. Clinical Endocrinology 60:420-428
593. Vierhapper H, Nowotny P, Waldhausl W (2003) Reduced production rates of testosterone and dihydrotestosterone in healthy men treated with rosiglitazone. Metabolism: Clinical and Experimental 52:230-232
594. Handelsman DJ, Mackey MA, Howe C, Turner L, Conway AJ (1997) Analysis of testosterone implants for androgen replacement therapy. Clinical Endocrinology 47:311-316
595. Kelleher S, Turner L, Howe C, Conway AJ, Handelsman DJ (1999) Extrusion of testosterone pellets: a randomized controlled clinical study. Clinical Endocrinology 51:469-471
596. Kelleher S, Conway AJ, Handelsman DJ (2002) A randomised controlled clinical trial of antibiotic impregnation of testosterone pellet implants to reduce extrusion rate. European Journal of Endocrinology 146:513-518
597. Kelleher S, Conway AJ, Handelsman DJ (2001) Influence of implantation site and track geometry on the extrusion rate and pharmacology of testosterone implants. Clinical Endocrinology 55:531-536
598. Cavender RK, Fairall M (2009) Subcutaneous testosterone pellet implant (Testopel) therapy for men with testosterone deficiency syndrome: a single-site retrospective safety analysis. J Sex Med 6:3177-3192
599. Pastuszak AW, Mittakanti H, Liu JS, Gomez L, Lipshultz LI, Khera M (2012) Pharmacokinetic evaluation and dosing of subcutaneous testosterone pellets. Journal of Andrology 33:927-937
600. McCullough AR, Khera M, Goldstein I, Hellstrom WJ, Morgentaler A, Levine LA (2012) A multi-institutional observational study of testosterone levels after testosterone pellet (Testopel((R))) insertion. J Sex Med 9:594-601
601. Bals-Pratsch M, Knuth UA, Yoon YD, Nieschlag E (1986) Transdermal testosterone substitution therapy for male hypogonadism. Lancet 2:943-946
602. Findlay JC, Place VA, Snyder PJ (1987) Transdermal delivery of testosterone. Journal of Clinical Endocrinology and Metabolism 64:266-268
603. Bals-Pratsch M, Langer K, Place VA, Nieschlag E (1988) Substitution therapy of hypogonadal men with transdermal testosterone over one year. Acta Endocrinologica 118:7-13
604. Jordan WP, Jr., Atkinson LE, Lai C (1998) Comparison of the skin irritation potential of two testosterone transdermal systems: an investigational system and a marketed product. Clinical Therapeutics 20:80-87
605. Jordan WP, Jr. (1997) Allergy and topical irritation associated with transdermal testosterone administration: a comparison of scrotal and nonscrotal transdermal systems. American Journal of Contact Dermatitis 8:108-113
606. Meikle AW, Mazer NA, Moellmer JF, Stringham JD, Tolman KG, Sanders SW, Odell WD (1992) Enhanced transdermal delivery of testosterone across nonscrotal skin produces physiological concentrations of testosterone and its metabolites in hypogonadal men. Journal of Clinical Endocrinology and Metabolism 74:623-628
607. Arver S, Dobs AS, Meikle AW, Caramelli KE, Rajaram L, Sanders SW, Mazer NA (1997) Long-term efficacy and safety of a permeation-enhanced testosterone transdermal system in hypogonadal men. Clinical Endocrinology 47:727-737
608. Shomaker TS, Zhang J, Ashburn MA (2001) A pilot study assessing the impact of heat on the transdermal delivery of testosterone. Journal of Clinical Pharmacology 41:677-682
609. Bennett NJ (1998) A burn-like lesion caused by a testosterone transdermal system. Burns 24:478-480
610. Wilson DE, Kaidbey K, Boike SC, Jorkasky DK (1998) Use of topical corticosteroid pretreatment to reduce the incidence and severity of skin reactions associated with testosterone transdermal therapy. Clinical Therapeutics 20:299-306
611. Guerin JF, Rollet J (1988) Inhibition of spermatogenesis in men using various combinations of oral progestagens and percutaneous or oral androgens. International Journal of Andrology 11:187-199
612. Fiet J, Morville R, Chemana D, Villette JM, Gourmel B, Brerault JL, Dreux C (1982) Percutaneous absorption of 5a-dihydrotestosterone in man. I Plasma androgen and gonadotrophin levels in normal adult men after percutaneous administration of 5a-dihydrotestosterone. International Journal of Andrology 5:586-594
613. Chemana D, Morville R, Fiet J, Villette JM, Tabuteau F, Brerault JL, Passa P (1982) Percutaneous absorption of 5a-dihydrotestosterone in man. II Percutaneous administration of 5a-dihydrotestosterone in hypogonadal men with idiopathic haemochromatosis; clinical, metabolic and hormonal effectiveness. International Journal of Andrology 5:595-606
614. Wang C, Iranmanesh A, Berman N, McDonald V, Steiner B, Ziel F, Faulkner SM, Dudley RE, Veldhuis JD, Swerdloff RS (1998) Comparative pharmacokinetics of three doses of percutaneous dihydrotestosterone gel in healthy elderly men--a clinical research center study. Journal of Clinical Endocrinology and Metabolism 83:2749-2757
615. Swerdloff RS, Wang C, Cunningham G, Dobs A, Iranmanesh A, Matsumoto AM, Snyder PJ, Weber T, Longstreth J, Berman N (2000) Long-term pharmacokinetics of transdermal testosterone gel in hypogonadal men. Journal of Clinical Endocrinology and Metabolism 85:4500-4510
616. Rolf C, Kemper S, Lemmnitz G, Eickenberg U, Nieschlag E (2002) Pharmacokinetics of a new transdermal testosterone gel in gonadotrophin-suppressed normal men. European Journal of Endocrinology 146:673-679
617. Steidle C, Schwartz S, Jacoby K, Sebree T, Smith T, Bachand R (2003) AA2500 testosterone gel normalizes androgen levels in aging males with improvements in body composition and sexual function. Journal of Clinical Endocrinology and Metabolism 88:2673-2681
618. McNicholas TA, Dean JD, Mulder H, Carnegie C, Jones NA (2003) A novel testosterone gel formulation normalizes androgen levels in hypogonadal men, with improvements in body composition and sexual function. BJU International 91:69-74
619. Kuhnert B, Byrne M, Simoni M, Kopcke W, Gerss J, Lemmnitz G, Nieschlag E (2005) Testosterone substitution with a new transdermal, hydroalcoholic gel applied to scrotal or non-scrotal skin: a multicentre trial. European Journal of Endocrinology 153:317-326
620. Wang C, Ilani N, Arver S, McLachlan RI, Soulis T, Watkinson A (2011) Efficacy and safety of the 2% formulation of testosterone topical solution applied to the axillae in androgen-deficient men. Clinical Endocrinology 75:836-843
621. Muram D, Melby T, Alles Kingshill E (2012) Skin reactions in a phase 3 study of a testosterone topical solution applied to the axilla in hypogonadal men. Current Medical Research and Opinion 28:761-766
622. Iyer R, Mok SF, Savkovic S, Turner L, Fraser G, Desai R, Jayadev V, Conway AJ, Handelsman DJ (2017) Pharmacokinetics of testosterone cream applied to scrotal skin. Andrology 5:725-731
623. Delanoe D, Fougeyrollas B, Meyer L, Thonneau P (1984) Androgenisation of female partners of men on medroxyprogesterone acetate/percutaneous testosterone contraception. Lancet i:276
624. Moore N, Paux G, Noblet C, Andrejak M (1988) Spouse-related drug side-effects. Lancet 1:468
625. de Ronde W (2009) Hyperandrogenism after transfer of topical testosterone gel: case report and review of published and unpublished studies. Human Reproduction 24:425-428
626. Yu YM, Punyasavatsu N, Elder D, D'Ercole AJ (1999) Sexual development in a two-year-old boy induced by topical exposure to testosterone. Pediatrics 104:e23
627. Bhowmick SK, Ricke T, Rettig KR (2007) Sexual precocity in a 16-month-old boy induced by indirect topical exposure to testosterone. Clinical Pediatrics 46:540-543
628. Brachet C, Vermeulen J, Heinrichs C (2005) Children's virilization and the use of a testosterone gel by their fathers. European Journal of Pediatrics 164:646-647
629. Svoren BM, Wolfsdorf JI (2005) Sexual development in a 21 month-old boy caused by testosterone contamination of a topical hydrocortisone cream. Journal of Pediatric Endocrinology and Metabolism 18:507-510
630. Kunz GJ, Klein KO, Clemons RD, Gottschalk ME, Jones KL (2004) Virilization of young children after topical androgen use by their parents. Pediatrics 114:282-284
631. Franklin SL, Geffner ME (2003) Precocious puberty secondary to topical testosterone exposure. Journal of Pediatric Endocrinology and Metabolism 16:107-110
632. Stahlman J, Britto M, Fitzpatrick S, McWhirter C, Testino SA, Brennan JJ, Zumbrunnen TL (2012) Serum testosterone levels in non-dosed females after secondary exposure to 1.62% testosterone gel: effects of clothing barrier on testosterone absorption. Current Medical Research and Opinion 28:291-301
633. Mazer N, Fisher D, Fischer J, Cosgrove M, Bell D, Eilers B (2005) Transfer of transdermally applied testosterone to clothing: a comparison of a testosterone patch versus a testosterone gel. J Sex Med 2:227-234
634. Stahlman J, Britto M, Fitzpatrick S, McWhirter C, Testino SA, Brennan JJ, Zumbrunnen TL (2012) Effect of application site, clothing barrier, and application site washing on testosterone transfer with a 1.62% testosterone gel. Current Medical Research and Opinion 28:281-290
635. Rolf C, Kemper S, Lemmnitz G, Eickenberg U, Nieschlag E (2002) Pharmacokinetics of a new transdermal testosterone gel in gonadotrophin-suppressed normal men. Eur J Endocrinol 146:673-679
636. de Ronde W, Vogel S, Bui HN, Heijboer AC (2011) Reduction in 24-hour plasma testosterone levels in subjects who showered 15 or 30 minutes after application of testosterone gel. Pharmacotherapy 31:248-252
637. Rolf C, Knie U, Lemmnitz G, Nieschlag E (2002) Interpersonal testosterone transfer after topical application of a newly developed testosterone gel preparation. Clin Endocrinol (Oxf) 56:637-641
638. Burris AS, Ewing LL, Sherins RJ (1988) Initial trial of slow-release testosterone microspheres in hypogonadal men. Fertility and Sterility 50:493-497
639. Bhasin S, Swerdloff RS, Steiner B, Peterson MA, Meridores T, Galmirini M, Pandian MR, Goldberg R, Berman N (1992) A biodegradable testosterone microcapsule formulation provides uniform eugonadal levels of testosterone for 10-11 weeks in hypogonadal men. Journal of Clinical Endocrinology and Metabolism 74:75-83
640. Amory JK, Anawalt BD, Blaskovich PD, Gilchriest J, Nuwayser ES, Matsumoto AM (2002) Testosterone release from a subcutaneous, biodegradable microcapsule formulation (Viatrel) in hypogonadal men. Journal of Andrology 23:84-91
641. Page ST, Bremner WJ, Clark RV, Bush MA, Zhi H, Caricofe RB, Smith PM, Amory JK (2008) Nanomilled oral testosterone plus dutasteride effectively normalizes serum testosterone in normal men with induced hypogonadism. Journal of Andrology 29:222-227
642. Amory JK, Bremner WJ (2005) Oral testosterone in oil plus dutasteride in men: a pharmacokinetic study. Journal of Clinical Endocrinology and Metabolism 90:2610-2617
643. Amory JK, Page ST, Bremner WJ (2006) Oral testosterone in oil: pharmacokinetic effects of 5alpha reduction by finasteride or dutasteride and food intake in men. Journal of Andrology 27:72-78
644. Johnsen SG, Kampmann JP, Bennet EP, Jorgensen F (1976) Enzyme induction by oral testosterone. Clinical Pharmacology and Therapeutics 20:233-237
645. Johnsen S (1978) Long-term oral testosterone and liver function. Lancet 1:50
646. Daggett PR, Wheeler MJ, Nabarro JD (1978) Oral testosterone, a reappraisal. Hormone Research 9:121-129
647. Lee A, Rubinow K, Clark RV, Caricofe RB, Bush MA, Zhi H, Roth MY, Page ST, Bremner WJ, Amory JK (2012) Pharmacokinetics of modified slow-release oral testosterone over 9 days in normal men with experimental hypogonadism. Journal of Andrology 33:420-426
648. Amory JK, Bush MA, Zhi H, Caricofe RB, Matsumoto AM, Swerdloff RS, Wang C, Clark RV (2011) Oral testosterone with and without concomitant inhibition of 5alpha-reductase by dutasteride in hypogonadal men for 28 days. Journal of Urology 185:626-632
649. Salehian B, Wang C, Alexander G, Davidson T, McDonald V, Berman N, Dudley RE, Ziel F, Swerdloff RS (1995) Pharmacokinetics, bioefficacy, and safety of sublingual testosterone cyclodextrin in hypogonadal men: comparison to testosterone enanthate--a clinical research center study. Journal of Clinical Endocrinology and Metabolism 80:3567-3575
650. Dobs AS, Hoover DR, Chen MC, Allen R (1998) Pharmacokinetic characteristics, efficacy, and safety of buccal testosterone in hypogonadal males: a pilot study. Journal of Clinical Endocrinology and Metabolism 83:33-39
651. Mazer N, Bell D, Wu J, Fischer J, Cosgrove M, Eilers B (2005) Comparison of the steady-state pharmacokinetics, metabolism, and variability of a transdermal testosterone patch versus a transdermal testosterone gel in hypogonadal men. J Sex Med 2:213-226
652. Swerdloff RS, Wang C (1998) Dihydrotestosterone: a rationale for its use as a non-aromatizable androgen replacement therapeutic agent. Baillieres Clinical Endocrinology and Metabolism 12:501-506
653. Junkman K (1957) Long-acting steroids in reproduction. Recent Progress in Hormone Research 13:380-419
654. Minto C, Howe C, Wishart S, Conway AJ, Handelsman DJ (1997) Pharmacokinetics and pharmacodynamics of nandrolone esters in oil vehicle: effects of ester, injection site and volume. Journal of Pharmacology and Experimental Therapeutics 281:93-102
655. Snyder PJ, Lawrence DA (1980) Treatment of male hypogonadism with testosterone enanthate. Journal of Clinical Endocrinology and Metabolism 51:1335-1339
656. Cantrill JA, Dewis P, Large DM, Newman M, Anderson DC (1984) Which testosterone replacement therapy? Clinical Endocrinology 24:97-107
657. Conway AJ, Boylan LM, Howe C, Ross G, Handelsman DJ (1988) A randomised clinical trial of testosterone replacement therapy in hypogonadal men. International Journal of Andrology 11:247-264
658. Behre HM, Wang C, Handelsman DJ, Nieschlag E 2004 Pharmacology of testosterone preparations. In: Nieschlag E, Behre HM eds. Testosterone: Action Deficiency Substitution. 3rd ed. Cambridge: Cambridge University Press; 405-444
659. Weinbauer GF, Marshall GR, Nieschlag E (1986) New injectable testosterone ester maintains serum testosterone of castrated monkeys in the normal range for four months. Acta Endocrinol 113:128-132
660. Behre HM, Nieschlag E (1992) Testosterone buciclate (20 Aet-1) in hypogonadal men: pharmacokinetics and pharmacodynamics of the new long-acting androgen ester. Journal of Clinical Endocrinology and Metabolism 75:1204-1210
661. Behre HM, Baus S, Kliesch S, Keck C, Simoni M, Nieschlag E (1995) Potential of testosterone buciclate for male contraception: endocrine differences between responders and nonresponders. Journal of Clinical Endocrinology and Metabolism 80:2394-2403
662. Zhang GY, Gu YQ, Wang XH, Cui YG, Bremner WJ (1998) A pharmacokinetic study of injectable testosterone undecanoate in hypogonadal men. Journal of Andrology 19:761-768
663. Nieschlag E, Buchter D, Von Eckardstein S, Abshagen K, Simoni M, Behre HM (1999) Repeated intramuscular injections of testosterone undecanoate for substitution therapy in hypogonadal men. Clinical Endocrinology 51:757-763.
664. von Eckardstein S, Nieschlag E (2002) Treatment of male hypogonadism with testosterone undecanoate injected at extended intervals of 12 weeks: a phase II study. Journal of Andrology 23:419-425
665. Schubert M, Minnemann T, Hubler D, Rouskova D, Christoph A, Oettel M, Ernst M, Mellinger U, Krone W, Jockenhovel F (2004) Intramuscular testosterone undecanoate: pharmacokinetic aspects of a novel testosterone formulation during long-term treatment of men with hypogonadism. Journal of Clinical Endocrinology and Metabolism 89:5429-5434
666. Gu YQ, Wang XH, Xu D, Peng L, Cheng LF, Huang MK, Huang ZJ, Zhang GY (2003) A multicenter contraceptive efficacy study of injectable testosterone undecanoate in healthy Chinese men. Journal of Clinical Endocrinology and Metabolism 88:562-568
667. Gu YQ, Tong JS, Ma DZ, Wang XH, Yuan D, Tang WH, Bremner WJ (2004) Male hormonal contraception: effects of injections of testosterone undecanoate and depot medroxyprogesterone acetate at eight-week intervals in chinese men. Journal of Clinical Endocrinology and Metabolism 89:2254-2262
668. Qoubaitary A, Meriggiola C, Ng CM, Lumbreras L, Cerpolini S, Pelusi G, Christensen PD, Hull L, Swerdloff RS, Wang C (2006) Pharmacokinetics of testosterone undecanoate injected alone or in combination with norethisterone enanthate in healthy men. Journal of Andrology 27:853-867
669. Mommers E, Kersemaekers WM, Elliesen J, Kepers M, Apter D, Behre HM, Beynon J, Bouloux PM, Costantino A, Gerbershagen HP, Gronlund L, Heger-Mahn D, Huhtaniemi I, Koldewijn EL, Lange C, Lindenberg S, Meriggiola MC, Meuleman E, Mulders PF, Nieschlag E, Perheentupa A, Solomon A, Vaisala L, Wu FC, Zitzmann M (2008) Male hormonal contraception: a double-blind, placebo-controlled study. Journal of Clinical Endocrinology and Metabolism 93:2572-2580
670. Jockenhovel F, Minnemann T, Schubert M, Freude S, Hubler D, Schumann C, Christoph A, Ernst M (2009) Comparison of long-acting testosterone undecanoate formulation versus testosterone enanthate on sexual function and mood in hypogonadal men. European Journal of Endocrinology 160:815-819
671. Singh GK, Turner L, Desai R, Jimenez M, Handelsman DJ (2014) Pharmacokinetic-pharmacodynamic study of subcutaneous injection of depot nandrolone decanoate using dried blood spots sampling coupled with ultrapressure liquid chromatography tandem mass spectrometry assays. J Clin Endocrinol Metab 99:2592-2598
672. Kaminetsky JC, McCullough A, Hwang K, Jaffe JS, Wang C, Swerdloff RS (2019) A 52-Week Study of Dose Adjusted Subcutaneous Testosterone Enanthate in Oil Self-Administered via Disposable Auto-Injector. J Urol 201:587-594
673. Olson J, Schrager SM, Clark LF, Dunlap SL, Belzer M (2014) Subcutaneous Testosterone: An Effective Delivery Mechanism for Masculinizing Young Transgender Men. LGBT Health 1:165-167
674. Spratt DI, Stewart, II, Savage C, Craig W, Spack NP, Chandler DW, Spratt LV, Eimicke T, Olshan JS (2017) Subcutaneous Injection of Testosterone Is an Effective and Preferred Alternative to Intramuscular Injection: Demonstration in Female-to-Male Transgender Patients. J Clin Endocrinol Metab 102:2349-2355
675. Wilson DM, Kiang TKL, Ensom MHH (2018) Pharmacokinetics, safety, and patient acceptability of subcutaneous versus intramuscular testosterone injection for gender-affirming therapy: A pilot study. Am J Health Syst Pharm 75:351-358
676. Turner L, Ly LP, Desai R, Singh GKS, Handelsman TD, Savkovic S, Fennell C, Jayadev V, Conway A, Handelsman DJ (2019) Pharmacokinetics and Acceptability of Subcutaneous Injection of Testosterone Undecanoate. J Endocr Soc 3:1531-1540
677. Kohn FM, Schill WB (2003) A new oral testosterone undecanoate formulation. World Journal of Urology 21:311-315
678. Bagchus WM, Hust R, Maris F, Schnabel PG, Houwing NS (2003) Important effect of food on the bioavailability of oral testosterone undecanoate. Pharmacotherapy 23:319-325
679. Schnabel PG, Bagchus W, Lass H, Thomsen T, Geurts TB (2007) The effect of food composition on serum testosterone levels after oral administration of Andriol Testocaps. Clinical Endocrinology 66:579-585
680. Roth MY, Dudley RE, Hull L, Leung A, Christenson P, Wang C, Swerdloff R, Amory JK (2011) Steady-state pharmacokinetics of oral testosterone undecanoate with concomitant inhibition of 5alpha-reductase by finasteride. International Journal of Andrology 34:541-547
681. Idan A, Griffiths KA, Harwood DT, Seibel MJ, Turner L, Conway AJ, Handelsman DJ (2010) Long-term effects of dihydrotestosterone treatment on prostate growth in healthy, middle-aged men without prostate disease: a randomized, placebo-controlled trial. Annals of Internal Medicine 153:621-632
682. Gooren LJ (1994) A ten-year safety study of the oral androgen testosterone undecanoate. Journal of Andrology 15:212-215.
683. Page ST, Lin DW, Mostaghel EA, Marck BT, Wright JL, Wu J, Amory JK, Nelson PS, Matsumoto AM (2011) Dihydrotestosterone administration does not increase intraprostatic androgen concentrations or alter prostate androgen action in healthy men: a randomized-controlled trial. Journal of Clinical Endocrinology and Metabolism 96:430-437
684. Tauber U, Schroder K, Dusterberg B, Matthes H (1986) Absolute bioavailability of testosterone after oral administration of testosterone-undecanoate and testosterone. European Journal of Drug Metabolism and Pharmacokinetics 11:145-149
685. Ahmed SF, Tucker P, Mayo A, Wallace AM, Hughes IA (2004) Randomized, crossover comparison study of the short-term effect of oral testosterone undecanoate and intramuscular testosterone depot on linear growth and serum bone alkaline phosphatase. Journal of Pediatric Endocrinology and Metabolism 17:941-950
686. Swerdloff RS, Wang C, White WB, Kaminetsky J, Gittelman MC, Longstreth JA, Dudley RE, Danoff TM (2020) A New Oral Testosterone Undecanoate Formulation Restores Testosterone to Normal Concentrations in Hypogonadal Men. J Clin Endocrinol Metab 105
687. Nieschlag E, Mauss J, Coert A, Kicovic P (1975) Plasma androgen levels in men after oral administration of testosterone or testosterone undecanoate. Acta Endocrinol (Copenh) 79:366-374
688. Butler GE, Sellar RE, Walker RF, Hendry M, Kelnar CJH, Wu FCW (1992) Oral testosterone undecanoate in the management of delayed puberty in boys: pharmacokinetics and effects on sexual maturation and growth. Journal of Clinical Endocrinology and Metabolism 75:37-44
689. Brown DC, Butler GE, Kelnar CJ, Wu FC (1995) A double blind, placebo controlled study of the effects of low dose testosterone undecanoate on the growth of small for age, prepubertal boys. Archives of Disease in Childhood 73:131-135
690. Albanese A, Kewley GD, Long A, Pearl KN, Robins DG, Stanhope R (1994) Oral treatment for constitutional delay of growth and puberty in boys: a randomised trial of an anabolic steroid or testosterone undecanoate. Archives of Disease in Childhood 71:315-317
691. Luisi M, Franchi E (1980) Double-blind group comparative study of testosterone undecanoate and mesterolone in hypogonadal male patients. Journal of Endocrinological Investigations 3:305-308
692. (1979) Androgen therapy of aplastic anaemia--a prospective study of 352 cases. Scandinavian Journal of Haematology 22:343-356
693. Shimoda K, Shide K, Kamezaki K, Okamura T, Harada N, Kinukawa N, Ohyashiki K, Niho Y, Mizoguchi H, Omine M, Ozawa K, Haradaa M (2007) The effect of anabolic steroids on anemia in myelofibrosis with myeloid metaplasia: retrospective analysis of 39 patients in Japan. International Journal of Hematology 85:338-343
694. Gennari C, AgnusDei D, Gonnelli S, Nardi P (1989) Effects of nandrolone decanoate therapy on bone mass and calcium metabolism in women with established post-menopausal osteoporosis: a double-blind placebo-controlled study. Maturitas 11:187-197
695. Frisoli A, Jr., Chaves PH, Pinheiro MM, Szejnfeld VL (2005) The effect of nandrolone decanoate on bone mineral density, muscle mass, and hemoglobin levels in elderly women with osteoporosis: a double-blind, randomized, placebo-controlled clinical trial. Journals of Gerontology Series A, Biological Sciences and Medical Sciences 60:648-653
696. Simpson ER, Mahendroo MS, Means GD, Kilgore MW, Hinshelwood MM, Graham-Lorence S, Amarneh B, Ito Y, Fisher CR, Michael MD, et al. (1994) Aromatase cytochrome P450, the enzyme responsible for estrogen biosynthesis. Endocrine Reviews 15:342-355
697. Hong Y, Yu B, Sherman M, Yuan YC, Zhou D, Chen S (2007) Molecular basis for the aromatization reaction and exemestane-mediated irreversible inhibition of human aromatase. Molecular Endocrinology 21:401-414
698. Hobbs CJ, Jones RE, Plymate SR (1996) Nandrolone, a 19-nortestosterone, enhances insulin-independent glucose uptake in normal men. Journal of Clinical Endocrinology and Metabolism 81:1582-1585
699. Behre HM, Kliesch S, Lemcke B, von Eckardstein S, Nieschlag E (2001) Suppression of spermatogenesis to azoospermia by combined administration of GnRH antagonist and 19-nortestosterone cannot be maintained by this non-aromatizable androgen alone. Human Reproduction 16:2570-2577
700. Attardi BJ, Pham TC, Radler LC, Burgenson J, Hild SA, Reel JR (2008) Dimethandrolone (7alpha,11beta-dimethyl-19-nortestosterone) and 11beta-methyl-19-nortestosterone are not converted to aromatic A-ring products in the presence of recombinant human aromatase. Journal of Steroid Biochemistry and Molecular Biology 110:214-222
701. Lemus AE, Enriquez J, Garcia GA, Grillasca I, Perez-Palacios G (1997) 5alpha-reduction of norethisterone enhances its binding affinity for androgen receptors but diminishes its androgenic potency. Journal of Steroid Biochemistry and Molecular Biology 60:121-129
702. Geusens P (1995) Nandrolone decanoate: pharmacological properties and therapeutic use in osteoporosis. Clinical Rheumatology 14:32-39.
703. Sundaram K, Kumar N (2000) 7alpha-methyl-19-nortestosterone (MENT): the optimal androgen for male contraception and replacement therapy. International Journal of Andrology 23 Suppl 2:13-15
704. Cook CE, Kepler JA (2005) 7alpha,11beta-Dimethyl-19-nortestosterone: a potent and selective androgen response modulator with prostate-sparing properties. Bioorganic and Medicinal Chemistry Letters 15:1213-1216
705. Suvisaari J, Sundaram K, Noe G, Kumar N, Aguillaume C, Tsong YY, Lahteenmaki P, Bardin CW (1997) Pharmacokinetics and pharmacodynamics of 7a-methyl-19-nortestosterone after intramuscular administration in healthy men. Human Reproduction 12:967-973
706. Sundaram K, Kumar N, Bardin CW (1994) 7 alpha-Methyl-19-nortestosterone: an ideal androgen for replacement therapy. Recent Progress in Hormone Research 49:373-376
707. Sundaram K, Kumar N, Bardin CW (1993) 7a-Methyl-nortestosterone (MENT): the optimal androgen for male contraception. Annals of Medicine 25:199-205
708. Attardi BJ, Hild SA, Reel JR (2006) Dimethandrolone undecanoate: a new potent orally active androgen with progestational activity. Endocrinology 147:3016-3026
709. Sundaram K, Kumar N, Monder C, Bardin CW (1995) Different patterns of metabolism determine the relative anabolic activity of 19-norandrogens. Journal of Steroid Biochemistry and Molecular Biology 53:253-257
710. Toth M, Zakar T (1982) Relative binding affinities of testosterone, 19-nortestosterone and their 5 alpha-reduced derivatives to the androgen receptor and to other androgen-binding proteins: a suggested role of 5 alpha-reductive steroid metabolism in the dissociation of "myotropic" and "androgenic" activities of 19-nortestosterone. Journal of Steroid Biochemistry 17:653-660
711. LaMorte A, Kumar N, Bardin CW, Sundaram K (1994) Aromatization of 7 alpha-methyl-19-nortestosterone by human placental microsomes in vitro. Journal of Steroid Biochemistry and Molecular Biology 48:297-304
712. Moslemi S, Dintinger T, Dehennin L, Silberzahn P, Gaillard JL (1993) Different in vitro metabolism of 7 alpha-methyl-19-nortestosterone by human and equine aromatases. European Journal of Biochemistry 214:569-576
713. Dobs AS, Boccia RV, Croot CC, Gabrail NY, Dalton JT, Hancock ML, Johnston MA, Steiner MS (2013) Effects of enobosarm on muscle wasting and physical function in patients with cancer: a double-blind, randomised controlled phase 2 trial. Lancet Oncology 14:335-345
714. Crawford J, Prado CM, Johnston MA, Gralla RJ, Taylor RP, Hancock ML, Dalton JT (2016) Study Design and Rationale for the Phase 3 Clinical Development Program of Enobosarm, a Selective Androgen Receptor Modulator, for the Prevention and Treatment of Muscle Wasting in Cancer Patients (POWER Trials). Current Oncology Reports 18:37
715. Yin D, Xu H, He Y, Kirkovsky LI, Miller DD, Dalton JT (2002) Pharmacology, pharmacokinetics, and metabolism of acetothiolutamide, a novel nonsteroidal agonist for the androgen receptor. Journal of Pharmacology and Experimental Therapeutics 304:1323-1333.
716. Gao W, Dalton JT (2007) Ockham's razor and selective androgen receptor modulators (SARMs): are we overlooking the role of 5alpha-reductase? Mol Interv 7:10-13
717. Sengupta S, Jordan VC (2008) Selective estrogen modulators as an anticancer tool: mechanisms of efficiency and resistance. Advances in Experimental Medicine and Biology 630:206-219
718. Gao W, Dalton JT (2007) Expanding the therapeutic use of androgens via selective androgen receptor modulators (SARMs). Drug Discov Today 12:241-248
719. Thole Z, Manso G, Salgueiro E, Revuelta P, Hidalgo A (2004) Hepatotoxicity induced by antiandrogens: a review of the literature. Urologia Internationalis 73:289-295
720. Manso G, Thole Z, Salgueiro E, Revuelta P, Hidalgo A (2006) Spontaneous reporting of hepatotoxicity associated with antiandrogens: data from the Spanish pharmacovigilance system. Pharmacoepidemiol Drug Saf 15:253-259
721. Minnemann T, Schubert M, Hubler D, Gouni-Berthold I, Freude S, Schumann C, Oettel M, Ernst M, Mellinger U, Sommer F, Krone W, Jockenhovel F (2007) A four-year efficacy and safety study of the long-acting parenteral testosterone undecanoate. Aging Male 10:155-158
722. Saad F, Kamischke A, Yassin A, Zitzmann M, Schubert M, Jockenhel F, Behre HM, Gooren L, Nieschlag E (2007) More than eight years' hands-on experience with the novel long-acting parenteral testosterone undecanoate. Asian Journal of Andrology 9:291-297
723. Stege R, Frohlander N, Carlstrom K, Pousette A, von Schoultz B (1987) Steroid-sensitive proteins, growth hormone and somatomedin C in prostatic cancer: effects of parenteral and oral estrogen therapy. Prostate 10:333-338
724. Serin IS, Ozcelik B, Basbug M, Aygen E, Kula M, Erez R (2001) Long-term effects of continuous oral and transdermal estrogen replacement therapy on sex hormone binding globulin and free testosterone levels. European Journal of Obstetrics, Gynecology, and Reproductive Biology 99:222-225
725. von Schoultz B, Carlstrom K (1989) On the regulation of sex-hormone-binding globulin. A challenge of an old dogma and outlines of an alternative mechanism. Journal of Steroid Biochemistry and Molecular Biology 32:327-334
726. Small M, Beastall GH, Semple CG, Cowan RA, Forbes CD (1984) Alterations of hormone levels in normal males given the anabolic steroid stanozolol. Clinical Endocrinology 21:49-55
727. Christiansen K 2004 Behavioural correlates of testosterone. In: Nieschlag E, Behre HM eds. Testosterone: Action Deficiency Substitution. 3rd ed. Cambridge: Cambridge University Press; 125-172
728. Anderson RA, Bancroft J, Wu FCW (1992) The effects of exogenous testosterone on sexuality and mood of normal men. Journal of Clinical Endocrinology and Metabolism 75:1503-1507
729. Buena F, Peterson MA, Swerdloff RS, Pandian MR, Steiner BS, Galmarini M, Lutchmansingh P, Bhasin S (1993) Sexual function does not change when serum testosterone levels are pharmacologically varied within the normal male range. Fertility and Sterility 59:1118-1123
730. Bagatell CJ, Heiman JR, Matsumoto AM, Rivier JE, Bremner WJ (1994) Metabolic and behavioral effects of high-dose, exogenous testosterone in healthy men. Journal of Clinical Endocrinology and Metabolism 79:561-567
731. Tricker R, Casaburi R, Storer TW, Clevenger B, Berman N, Shirazi A, Bhasin S (1996) The effects of supraphysiological doses of testosterone on angry behavior in healthy eugonadal men--a clinical research center study. Journal of Clinical Endocrinology and Metabolism 81:3754-3758
732. O'Connor DB, Archer J, Hair WM, Wu FC (2002) Exogenous testosterone, aggression, and mood in eugonadal and hypogonadal men. Physiology and Behavior 75:557-566
733. O'Connor DB, Archer J, Wu FC (2004) Effects of testosterone on mood, aggression, and sexual behavior in young men: a double-blind, placebo-controlled, cross-over study. Journal of Clinical Endocrinology and Metabolism 89:2837-2845
734. Meriggiola MC, Cerpolini S, Bremner WJ, Mbizvo MT, Vogelsong KM, Martorana G, Pelusi G (2006) Acceptability of an injectable male contraceptive regimen of norethisterone enanthate and testosterone undecanoate for men. Human Reproduction 21:2033-2040
735. Su TP, Pagliaro M, Schmidt PJ, Pickar D, Wolkowitz O, Rubinow DR (1993) Neuropsychiatric effects of anabolic steroids in male normal volunteers. Journal of the American Medical Association 269:2760-2764
736. Yates WR, Perry PJ, MacIndoe J, Holman T, Ellingrod V (1999) Psychosexual effects of three doses of testosterone cycling in normal men. Biological Psychiatry 45:254-260
737. Daly RC, Su TP, Schmidt PJ, Pagliaro M, Pickar D, Rubinow DR (2003) Neuroendocrine and behavioral effects of high-dose anabolic steroid administration in male normal volunteers. Psychoneuroendocrinology 28:317-331
738. Schmidt PJ, Berlin KL, Danaceau MA, Neeren A, Haq NA, Roca CA, Rubinow DR (2004) The effects of pharmacologically induced hypogonadism on mood in healthy men. Archives of General Psychiatry 61:997-1004
739. WHO Task Force on Methods for the Regulation of Male Fertility (1996) Contraceptive efficacy of testosterone-induced azoospermia and oligozoospermia in normal men. Fertility and Sterility 65:821-829
740. WHO Task Force on Methods for the Regulation of Male Fertility (1990) Contraceptive efficacy of testosterone-induced azoospermia in normal men. Lancet 336:955-959
741. Bjorkvist K, Nygren T, Bjorklund AC, Bjorkvist SE (1994) Testosterone intake and aggressiveness: real effect or anticipation? Aggressive Behavior 20:17-26
742. Archer J (1991) The influence of testosterone on human aggression. British Journal of Psychiatry 82:1-28
743. Melnik B, Jansen T, Grabbe S (2007) Abuse of anabolic-androgenic steroids and bodybuilding acne: an underestimated health problem. J Dtsch Dermatol Ges 5:110-117
744. Grunstein RR, Handelsman DJ, Lawrence SJ, Blackwell C, Caterson ID, Sullivan CE (1989) Hypothalamic dysfunction in sleep apnea: reversal by nasal continuous positive airways pressure. Journal of Clinical Endocrinology and Metabolism 68:352-358
745. Sandblom RE, Matsumoto AM, Scoene RB, Lee KA, Giblin EC, Bremner WJ, Pierson DJ (1983) Obstructive sleep apnea induced by testosterone administration. New England Journal of Medicine 308:508-510
746. Liu PY, Yee BJ, Wishart SM, Jimenez M, Jung DG, Grunstein RR, Handelsman DJ (2003) The short-term effects of high dose testosterone on sleep, breathing and function in older men. Journal of Clinical Endocrinology and Metabolism 88:3605-3613
747. Martinez C, Suissa S, Rietbrock S, Katholing A, Freedman B, Cohen AT, Handelsman DJ (2016) Testosterone treatment and risk of venous thromboembolism: population based case-control study. BMJ 355:i5968
748. Glueck CJ, Goldenberg N, Wang P (2018) Thromboembolism peaking 3 months after starting testosterone therapy: testosterone-thrombophilia interactions. J Investig Med 66:733-738
749. Glueck CJ, Goldenberg N, Budhani S, Lotner D, Abuchaibe C, Gowda M, Nayar T, Khan N, Wang P (2011) Thrombotic events after starting exogenous testosterone in men with previously undiagnosed familial thrombophilia. Transl Res 158:225-234
750. Walker RF, Zakai NA, MacLehose RF, Cowan LT, Adam TJ, Alonso A, Lutsey PL (2019) Association of Testosterone Therapy With Risk of Venous Thromboembolism Among Men With and Without Hypogonadism. JAMA Intern Med 180:190-197
751. Baillargeon J, Urban RJ, Morgentaler A, Glueck CJ, Baillargeon G, Sharma G, Kuo YF (2015) Risk of Venous Thromboembolism in Men Receiving Testosterone Therapy. Mayo Clin Proc 90:1038-1045
752. Li H, Benoit K, Wang W, Motsko S (2016) Association between Use of Exogenous Testosterone Therapy and Risk of Venous Thrombotic Events among Exogenous Testosterone Treated and Untreated Men with Hypogonadism. J Urol 195:1065-1072
753. Cole AP, Hanske J, Jiang W, Kwon NK, Lipsitz SR, Kathrins M, Learn PA, Sun M, Haider AH, Basaria S, Trinh QD (2018) Impact of testosterone replacement therapy on thromboembolism, heart disease and obstructive sleep apnoea in men. BJU Int 121:811-818
754. Houghton DE, Alsawas M, Barrioneuvo P, Tello M, Farah W, Beuschel B, Prokop LJ, Layton JB, Murad MH, Moll S (2018) Testosterone therapy and venous thromboembolism: A systematic review and meta-analysis. Thromb Res 172:94-103
755. Glueck CJ, Goldenberg N, Wang P (2018) Testosterone Therapy, Thrombophilia, Venous Thromboembolism, and Thrombotic Events. J Clin Med 8
756. Luo S, Au Yeung SL, Zhao JV, Burgess S, Schooling CM (2019) Association of genetically predicted testosterone with thromboembolism, heart failure, and myocardial infarction: mendelian randomisation study in UK Biobank. Bmj 364:l476
757. Svartberg J, Braekkan SK, Laughlin GA, Hansen JB (2009) Endogenous sex hormone levels in men are not associated with risk of venous thromboembolism: the Tromso study. Eur J Endocrinol 160:833-838
758. Welder AA, Robertson JW, Melchert RB (1995) Toxic effects of anabolic-androgenic steroids in primary rat hepatic cell cultures. Journal of Pharmacological and Toxicological Methods 33:187-195
759. Gitlin N, Korner P, Yang HM (1999) Liver function in postmenopausal women on estrogen-androgen hormone replacement therapy: a meta-analysis of eight clinical trials. Menopause 6:216-224
760. Gelfand MM, Wiita B (1997) Androgen and estrogen-androgen hormone replacement therapy: a review of the safety literature, 1941 to 1996. Clinical Therapeutics 19:383-404; discussion 367-388
761. Pertusi R, Dickerman RD, McConathy WJ (2001) Evaluation of aminotransferase elevations in a bodybuilder using anabolic steroids: hepatitis or rhabdomyolysis? Journal of the American Osteopathic Association 101:391-394
762. Tsokos M, Erbersdobler A (2005) Pathology of peliosis. Forensic Science International 149:25-33
763. Falk H, Thomas LB, Popper H, Ishak KG (1979) Hepatic angiosarcoma associated with androgenic-anabolic steroids. Lancet 2:1120-1123
764. Daneshmend TK, Bradfield JW (1979) Hepatic angiosarcoma associated with androgenic-anabolic steroids. Lancet 2:1249
765. Mackey MA, Conway AJ, Handelsman DJ (1995) Tolerability of intramuscular injections of testosterone ester in an oil vehicle. Human Reproduction 10:862-865
766. Bhagat R, Holmes IH, Kulaga A, Murphy F, Cockcroft DW (1995) Self-injection with olive oil. A cause of lipoid pneumonia. Chest 107:875-876
767. Hjort M, Hoegberg LC, Almind M, Jansen T (2015) Subacute fat-embolism-like syndrome following high-volume intramuscular and accidental intravascular injection of mineral oil. Clin Toxicol (Phila) 53:230-232
768. Middleton T, Turner L, Fennell C, Savkovic S, Jayadev V, Conway AJ, Handelsman DJ (2015) Complications of injectable testosterone undecanoate in routine clinical practice. European Journal of Endocrinology 172:511-517
769. Meyer RJ, Mann M (2015) Pulmonary oil micro-embolism (POME) syndrome: a review and summary of a large case series. Current Medical Research and Opinion 31:837-841
770. Darsow U, Bruckbauer H, Worret WI, Hofmann H, Ring J (2000) Subcutaneous oleomas induced by self-injection of sesame seed oil for muscle augmentation. Journal of the American Academy of Dermatology 42:292-294
771. Lawrentschuk N, Fleshner N (2009) Severe irritant contact dermatitis causing skin ulceration secondary to a testosterone patch. TheScientificWorldJournal 9:333-338
772. Rolf C, Knie U, Lemmnitz G, Nieschlag E (2002) Interpersonal testosterone transfer after topical application of a newly developed testosterone gel preparation. Clinical Endocrinology 56:637-641
773. Zitzmann M, Faber S, Nieschlag E (2006) Association of specific symptoms and metabolic risks with serum testosterone in older men. Journal of Clinical Endocrinology and Metabolism 91:4335-4343
774. Jockenhovel F, Minnemann T, Schubert M, Freude S, Hubler D, Schumann C, Christoph A, Gooren L, Ernst M (2009) Timetable of effects of testosterone administration to hypogonadal men on variables of sex and mood. Aging Male 12:113-118
775. Mooradian AD, Morley JE, Korenman SG (1987) Biological actions of androgens. Endocrine Reviews 8:1-28
776. Palacios A, Campfield LA, McClure RD, Steiner B, Swerdloff RS (1983) Effect of testosterone enanthate on hematopoesis in normal men. Fertility and Sterility 40:100-104
777. Drinka PJ, Jochen AL, Cuisinier M, Bloom R, Rudman I, Rudman D (1995) Polycythemia as a complication of testosterone replacement therapy in nursing home men with low testosterone levels. Journal of the American Geriatric Society 43:899-901
778. Ip FF, di Pierro I, Brown R, Cunningham I, Handelsman DJ, Liu PY (2010) Trough serum testosterone predicts the development of polycythemia in hypogonadal men treated for up to 21 years with subcutaneous testosterone pellets. European Journal of Endocrinology 162:385-390
779. Viallard JF, Marit G, Mercie P, Leng B, Reiffers J, Pellegrin JL (2000) Polycythaemia as a complication of transdermal testosterone therapy. British Journal of Haematology 110:237-238
780. Tefferi A (2012) Polycythemia vera and essential thrombocythemia: 2012 update on diagnosis, risk stratification, and management. American Journal of Hematology 87:285-293
781. Siddique H, Smith JC, Corrall RJ (2004) Reversal of polycythaemia induced by intramuscular androgen replacement using transdermal testosterone therapy. Clinical Endocrinology 60:143-145
782. Wu JP, Gu FL (1987) The prostate 41-65 years post castration. Chinese Medical Journal 100:271-272
783. Swerdlow AJ, Schoemaker MJ, Higgins CD, Wright AF, Jacobs PA (2005) Cancer incidence and mortality in men with Klinefelter syndrome: a cohort study. Journal of the National Cancer Institute 97:1204-1210
784. Behre HM, Bohmeyer J, Nieschlag E (1994) Prostate volume in testosterone-treated and untreated hypogonadal men in comparison to age-matched normal controls. Clinical Endocrinology 40:341-349
785. Jin B, Conway AJ, Handelsman DJ (2001) Effects of androgen deficiency and replacement on prostate zonal volumes. Clinical Endocrinology 54:437-445.
786. Handelsman DJ 1998 The safety of androgens: prostate and cardiovascular disease. In: Wang C ed. Male Reproductive Function. Boston: Kluwer Academic Publishers; 173-190
787. Fowler JE, Jr., Whitmore WF, Jr. (1981) The response of metastatic adenocarcinoma of the prostate to exogenous testosterone. Journal of Urology 126:372-375
788. Fowler JE, Jr., Whitmore WF, Jr. (1982) Considerations for the use of testosterone with systemic chemotherapy in prostatic cancer. Cancer 49:1373-1377
789. Wright JL, Higano CS, Lin DW (2006) Intermittent androgen deprivation: clinical experience and practical applications. Urologic Clinics of North America 33:167-179, vi
790. Feltquate D, Nordquist L, Eicher C, Morris M, Smaletz O, Slovin S, Curley T, Wilton A, Fleisher M, Heller G, Scher HI (2006) Rapid androgen cycling as treatment for patients with prostate cancer. Clinical Cancer Research 12:7414-7421
791. Manni A, Bartholomew M, Caplan R, Boucher A, Santen R, Lipton A, Harvey H, Simmonds M, White-Hershey D, Gordon R, et al. (1988) Androgen priming and chemotherapy in advanced prostate cancer: evaluation of determinants of clinical outcome. Journal of Clinical Oncology 6:1456-1466
792. Santen RJ, Manni A, English HF, Heitjan D (1990) Androgen-primed chemotherapy-experimental confirmation of efficacy. Journal of Steroid Biochemistry and Molecular Biology 37:1115-1120
793. Szmulewitz R, Mohile S, Posadas E, Kunnavakkam R, Karrison T, Manchen E, Stadler WM (2009) A randomized phase 1 study of testosterone replacement for patients with low-risk castration-resistant prostate cancer. European Urology 56:97-103
794. Morris MJ, Huang D, Kelly WK, Slovin SF, Stephenson RD, Eicher C, Delacruz A, Curley T, Schwartz LH, Scher HI (2009) Phase 1 trial of high-dose exogenous testosterone in patients with castration-resistant metastatic prostate cancer. European Urology 56:237-244
795. Cui Y, Zong H, Yan H, Zhang Y (2014) The effect of testosterone replacement therapy on prostate cancer: a systematic review and meta-analysis. Prostate Cancer Prostatic Dis 17:132-143
796. Kaufman JM, Graydon RJ (2004) Androgen replacement after curative radical prostatectomy for prostate cancer in hypogonadal men. Journal of Urology 172:920-922
797. Rhoden EL, Averbeck MA, Teloken PE (2008) Androgen replacement in men undergoing treatment for prostate cancer. J Sex Med 5:2202-2208
798. Morgentaler A (2009) Testosterone therapy in men with prostate cancer: scientific and ethical considerations. Journal of Urology 181:972-979
799. (1971) Hormones in advanced cancer. British Medical Journal 2:760-763
800. Muggia FM (1990) Overview of cancer-related hypercalcemia: epidemiology and etiology. Seminars in Oncology 17:3-9