ABSTRACT
In mammals and man, historical investigation suggests that early recognition for a role of the hypothalamus as a site for integration of endocrine, autonomic and behavioral responses can be dated to the 2nd -18th centuries A.D. Although the hypothalamus comprises only 2% of the total brain volume, it is a key regulator of pituitary function and homeostatic balance. In this chapter, we provide an overview of the historical landmarks, embryologic, gross, microscopic and functional anatomy of the mammalian and human hypothalamus and pituitary, and how the hypothalamus relates to the rest of the brain and responds to peripheral signals. In particular, we show that its rostral, nuclear portion exerts prominent regulation of homeostatic behaviors related to energy balance and reproduction. The two caudal portions are primarily involved in ensuring adequate metabolic resources for defensive and exploratory behaviors and responses to sudden changes in endogenous and exogenous stimuli. In addition, we discuss how its network of neurons is made of cells with different functions (neurosecretory, autonomic, motor), how they interact, and how these neural circuitries are woven into a complex architecture of conduits for the movement of intercellular fluids (vasculature, glymphatic channels, meningeal lymphatic vessels). Finally, we focus on the hypothalamic mechanisms involved in the regulation of anterior and posterior pituitary secretion (hypothalamic tuberoinfundibular and neurohypophysial systems), as well those involved in food and fluid intake, lactation, thermoregulation, circadian rhythmicity and the sleep-wake cycle.
HISTORICAL OVERVIEW
As suggested by its Greek derivation, the hypothalamus (hypo = below, thalamus = bed) is that portion of the diencephalon in all vertebrates that lies inferior to the thalamus (1). The hypothalamus and pituitary gland have attracted the interest of scientists and artists for centuries since the first description by Galen of Pergamon in the 2nd century AD. Galen described the hypothalamic infundibulum and the pituitary gland in De Usu Partium as the draining route and receptacle, respectively, for mucus passing from the brain ventricular structures (primarily the third ventricle) to the nasopharynx, and named the capillary network surrounding the pituitary gland the rete mirabilis (2). Notably, he also recognized the association of the third ventricle with a dorsally-located, small gland he named “pineal”. The Galenic concepts dominated scientific thought about the hypothalamus and pituitary for approximately 1200 years until the 14th century when the Italian anatomist, Mondino de’ Liuzzi, in his Anothomia, proposed that the third ventricle serves as an “integrator” of body functions (Fig. 1) (3). Some of these ideas were extended by Andreas Vesalius in the 16th century in De Humani Corporis Fabrica, the first anatomical depiction of the infudibular-pituitary stalk including part of their venous drainage, consistent with our current anatomical knowledge for petrosal sinus sampling (Fig. 2). Attention to the importance of the hypothalamic-pituitary region influenced the work of some of the most famous Renaissance artists including Leonardo da Vinci, whose drawings of the third ventricle and rete mirabilis are shown in Fig. 3, and Michelangelo Buonarroti, whose painting on the ceiling of the Sistine Chapel in the Vatican uses the brain including the hypothalamic-pituitary region as a backdrop to his depiction of the creation of man (Fig. 4) (4). Further interest in the functional role of the third ventricle occurred during the 17th century by the philosopher, Renè Descartes. He hypothesized that a photic stimulus might reach the pineal gland from the retina, passing through the optic chiasm and third ventricle to stimulate the somatic motor nerves destined to the peripheral muscles to produce movement (Fig. 5).
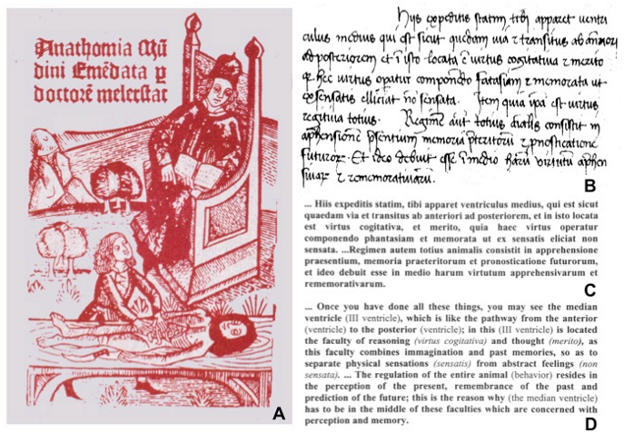
Figure. 1. Description of the functional role exerted by the cerebral third ventricle, as reported by Mondino de’ Liuzzi in Anothomia. (A) Original front page of Anothomia in a XIV century edition; (B) Original text (in brackets) in medieval Latin (from the 1316 A.D. manuscript kept at the Società Medica Chirurgica in Bologna, Italy); (C) a portion of the Latin fragment shown in (B) containing the most important concepts; (D) English translation shown in (B). (From Toni R., Ancient views on the hypothalamic-pituitary-thyroid axis: an historical and epistemological perspective, Pituitary 3: 83-95, 2000).
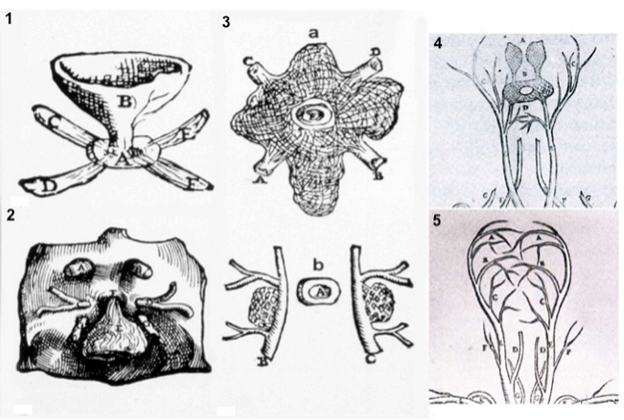
Figure 2. Plates from the seventh book of the first edition (1543) of the Fabrica by Andreas Vesalius, showing what is believed to be the oldest anatomical drawings in Western literature of the hypothalamic-pituitary unit. (Courtesy of the Library of the Department of Human Anatomy of the University of Bologna, Italy, with permission) 1) Enlarged view of the pituitary gland (A), hypothalamic infundibulum (B) and ducts comprising the foramen lacerum and superior orbital fissure (C, D, E, F) believed to drain brain mucus or phlegm (in Latin pituita) from the pituitary gland to the nasopharynx; 2) anatomical relationships between the infundibulum (E), the dural diaphragma sellae (F), the internal carotid arteries (C, D) and oculomotor nerves (G), all seen from above and, thus ventral to the posterior clinoid processes of the sella turcica (A, B); 3) composite image including a) an enlarged view of the rete mirabilis formed as a reticular plexus by the carotid arteries entering (A, B) and emerging (C, D) around the pituitary gland (E); b) detailed view of the reticular plexus arising from the carotids (B, C) on each side of the pituitary (A); 4) anatomy of the arterial, vertebral (dorsal vessels, F) and common carotid (ventral vessels, E) systems: the rete mirabilis (B) is provided by the internal carotid artery (D), branching medially with respect to the external carotid artery (C). Note that Vesalius portrayed the rete mirabilis widening symmetrically and superiorly (A) to vascularize the area of the infundibulum and hypothalamic floor, anticipating our current knowledge of the circuminfundibular and prechiasmal arteriolar-capillary plexus; 5) anatomy of the venous vertebral (D) and internal jugular (C) systems, including the common facial vein (D). Note the X-shaped, venous pattern at the center of the image, pointing to the area of the rete mirabilis: it is provided by four symmetrical branches of the internal jugular vein, and recapitulates the distribution of the inferior and superior petrosal, and spheno-parietal sinuses around the cavernous sinus. Thus, this drawing can be considered the first demonstration of a venous route from the pituitary through the internal jugular system, exploited for sampling of pituitary hormonal secretions only in the 2nd half of the 20th century. (From Toni R., Ancient views on the hypothalamic-pituitary-thyroid axis: an historical and epistemological perspective, Pituitary 3: 83-95, 2000, and Toni R. “Il sistema ipotalamo-ipofisario nell’antichità [The hypothalamic-pituitary system in the antiquity] - Dedicato alla memoria del Prof. Aldo Pinchera [Dedicated to the memory of Prof. Aldo Pinchera], In: L’Endocrinologo, Per una Storia dell’Endocrinologia [For a History of Endocrinology], 13, suppl. to n. 6, 1-11, 2012.
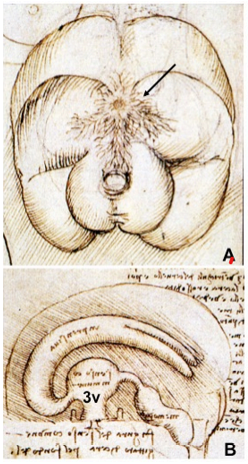
Figure 3. Drawings by Leonardo da Vinci (1508-1509) taken from the Codici di Anatomia of the Windsor’s Collection (Courtesy of the Library of the Department of Human Anatomy of the University of Parma, Italy). (A) Inferior surface of the brain, showing the rete mirabilis (arrow) that surrounds the pituitary gland; (B) three-dimensional representation of the cerebral ventricles. The third ventricle (3v) was believed to be the site of afference and elaboration of the “sensus communis” (Latin for peripheral physical sensations). (From Toni R, Malaguti A, Benfenati F, Martini L: The human hypothalamus: a morphofunctional perspective. J Endocrinol Invest 27 (supp to n.6), 73-94, 2004.)
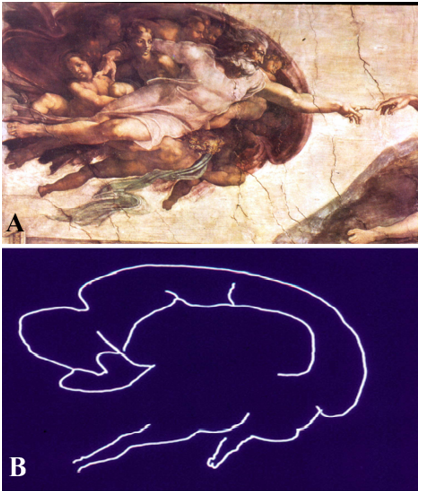
Figure 4. Detail from the fresco, “Creation of Adam,” by Michelangelo Buonarroti, visible on the ceiling of the Sistine Chapel in the Vatican at Rome, Italy, painted between 1508-1512. (A) Photograph of the fresco showing God giving spiritual life and intellect to Adam through his touch; (B) The contour of the same image is reminiscent of a midline sagittal section of the brain and includes the hypothalamus, pituitary and brainstem. (From Toni R, Malaguti A, Benfenati F, Martini L: The human hypothalamus: a morphofunctional perspective. J Endocrinol Invest 27 (supp to n.6), 73-94, 2004.)
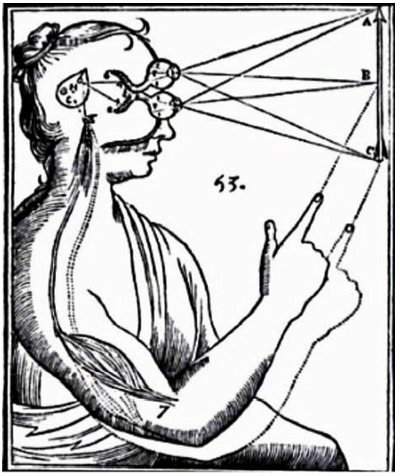
Figure 5. Drawing from the De Homine of Descartes (1662), showing the pathway of the light through the ocular globe, retina, and collaterals of the optic nerves (corresponding to the retino-hypothalamic tract) that project to the 3rd ventricle (i.e., to the suprachiasmatic nucleus – SCN), to stimulate the pineal gland to release the animal spirit (corresponding to the nerve impulse) to the peripheral striatal muscles. Indeed, we currently know that the photic stimuli may not only activate a hypothalamic-medullary-epithalamic pathway for melatonin release (see Circadian Rhythm section) and thus signal the day/night shift to SCN-dependent pituitary secretions, but also trigger the lateral habenular nucleus. The lateral habenular nucleus receives projections from the SCN directly through the stria medullaris of the thalamus, and indirectly via the superior colliculi by lateral hypothalamic efferents. It influences brainstem motor centers like the substantia nigra and reticular raphe nuclei, to regulate body movement in relation to visual clues. In addition, melatonin acts on skeletal muscle as an ergogenic factor, favoring aerobic motor performance. Thus, Descartes view of the hypothalamic-pineal connection is partly consistent with evidence that light impulses transmitted through the hypothalamus may influence motor activity via the pineal gland and related epithalamus. (From Toni R. “Il sistema ipotalamo-ipofisario nell’antichità [The hypothalamic-pituitary system in the antiquity] - Dedicato alla memoria del Prof. Aldo Pinchera [Dedicated to the memory of Prof. Aldo Pinchera], In: L’Endocrinologo, Per una Storia dell’Endocrinologia [For a History of Endocrinology], 13, suppl. to n. 6, 1-11, 2012.
The current term “hypothalamus", however, was not actually introduced until 1893 by the Swiss anatomist, Wilhelm His. On the basis of his studies on the ontogenesis of the human, fetal brain, His named the first anatomical subdivision of the hypothalamus the “pars optica hypothalami” (5), which is now recognized to include the preoptic region, tuber cinerium and infundibulum. Discovery of the connection between the hypothalamus and posterior pituitary (supraoptic-hypophysial tract) by Ramon Cajal in 1894, and subsequent work on neurosecretion in fish hypothalamus by the Sharrers in 1928, set the groundwork for rapid advancement in the understanding of the hypothalamus that unraveled throughout the 20th century and continues into the 21st century. Table 1 summarizes the major historical advances in the elucidation of the functional anatomy of the mammalian hypothalamic-pituitary unit (6).
Table 1. Timeline of Major Breakthroughs in Elucidation of the Functional Anatomy of the Mammalian Hypothalamic-Pituitary Unit
|
II century A.D.
|
Galen in Anatomicae Administrationes describes the third ventricle and its association with the rete mirabilis around the pituitary gland and dorsally with the pineal gland. In De Usum Partium considers the hypothalamic infundibulum and pituitary gland as draining route and receptacle for brain mucous to the nasopharynx
|
1928
|
E. Scharrer describes “glandular cells” in the fish hypothalamus (concept of “neurosecretion”)
|
1316
|
Mondino de Liuzzi da Bologna in his Anothomia refers to the third cerebral ventricle as “integrator” of body functions, including psychic, emotional, and behavioral responses
|
1930
|
Popa and Fielding describe in the human pituitary stalk a portal vascular system interpreted as a route of the blood upward the hypothalamus
|
1522
|
Berangario da Carpi in his Isagogue Breves denies the existence of the Galenic rete mirabilis in the human brain
|
1940-1955
|
Harris and Green establish the basis for the neural control of the pituitary gland secretion and demonstrate its vascular link with the hypothalamus
|
1543
|
Vesalius includes in the Fabricathe first anatomical drawings of the hypothalamic infundibulum, pituitary and their venous drainage
|
1954
|
WH Hess shows that both pituitary and autonomic responses are regulated by the anterior (trophotropic area) and posterior (ergotropic area) hypothalamus
|
1561-1627
|
Fallopius in the Observationes Anatomicae and Casserio in the Tabulae Anatomicaemention the arterial polygon at the base of the brain then described by Willis
|
1950-1958
|
Nauta and Kuypers describe the connections of the mammalian hypothalamus with the rest of the brain and propose that the limbic system influences pituitary function, introducing the concept of “hypothalamic integration”
|
1662
|
Descartes in his De Hominesuggests a connection between the optic nerve, third ventricle, and pineal gland to regulate body mouvments and coupling between neuroendocrine and motor responses in hypothalamic motivated behaviors
|
1960
|
Martinez describes the structure of the median eminence
|
1664
|
Willis in his Cerebri Anatomeargues that humors out of the third ventricle may be carried to the pituitary gland
|
1962
|
Halaz put forth the concept of “hypophysiotrophic area” of the hypothalamus
|
1655-1672
|
Schneider and Lower reject the Galenic idea that the pituitary gland filters brain secretions to the nose
|
1964
|
Szentagothi defines the tuberoinfundibular tract
|
1742
|
Lieutand discovers vessels in the pituitary stalk
|
1968
|
Guillemin and Schally isolate the first hypothalamic releasing factor
|
1767
|
Luigi Galvani in Disquisitiones Anatomicae circa Membranam Pituitariam discovers that mucus passing through the nostrils originates from small mucous glands of the human nasal mucosa and not from the pituitary
|
1969-1970
|
Yoshimura et al. show that mice pituitary chromophobes may behave like pituitary stem cells, and Nakane provides the first ultrastructural evidence for paracrine interactions in the pituitary gland
|
1778
|
Sommering introduces the term “hypophysis”
|
1971
|
L. Martini shows that hypothalamic releasing-factors regulate their own secretion via an “ultrashort feedback”
|
1787
|
Paolo Mascagni describes lymphatic vessels in human cranial meninges, introducing the modern view of a lymphatic drainage of brain structures in mammals and man
|
1984
|
T. Hokfelt demonstrates the presence of two different neurotransmitters in the same hypothalamic neuron, introducing the concept of “neuroendocrine regulation by multiple neuronal messengers”
|
1860
|
Von Luska describes the primary (or hypothalamic) capillary plexus of the portal vessels
|
1986
|
K. Fuxe and L. F. Agnati show that the median eminence is organized in modules, introducing the concept of “medianosome”, and hypothalamic neurons are regulated by both autocrine/paracrine and synaptic mechanisms, better known as “volume and wiring transmissions”
|
1872-1877
|
Meynert and Forel define the anatomical borders of what they call “the neural portion extending forward the region of the subthalamus” (i.e., the hypothalamus)
|
2009
|
Garcia-Lavandeira et al. identify stem cells/progenitors in the marginal zone of the adult human pituitary gland
|
1893
|
His introduces the term “hypothalamus” and provides the first anatomical subdivision based on ontogenesis of the human brain
|
2012
|
Iliff et al. describe the “glymphatic system” in rodents, glia-dependent perivascular tunnels interconnected with meningeal lymphatics, allowing for distribution of metabolites and neuromodulators to hypothalamic neurons
|
|
|
|
|
(Based on Toni R, Malaguti A, Benfenati F, Martini L: The human hypothalamus: a morphofunctional perspective. J Endocrinol Invest 27, supp to n.6, 73-94, 2004, and Toni R. “Il sistema ipotalamo-ipofisario nell’antichità [The hypothalamic-pituitary system in the antiquity] - Dedicato alla memoria del Prof. Aldo Pinchera [Dedicated to the memory of Prof. Aldo Pinchera], In: L’Endocrinologo, Per una Storia dell’Endocrinologia [For a History of Endocrinology], 13, suppl. to n. 6, 1-11, 2012
ANATOMY OF THE PITUITARY GLAND
Gross and Radiologic Anatomy
The pituitary gland lies within a recess of the median part of the middle cranial fossa in the sphenoid bone (sella turcica) and is composed of two major components, the anterior lobe (adenohypophysis) and the posterior lobe (neurohypophysis) that can be readily distinguished radiologically by magnetic resonance imaging (Fig. 6). The anterior lobe contains three subdivisions including the pars distalis, pars intermedia and pars tuberalis. The pars distalis makes up the bulk of the anterior pituitary and is responsible for the secretion of anterior pituitary hormones into the peripheral circulation. In man, it contains follicles of different sizes, typically surrounded by folliculostellate (FS) cells (7). The pars intermedia lies between the pars distalis and the posterior pituitary, representing what remains of the original Rathke’s pouch cleft (see section on Embyryologic Anatomy). Although considered vestigial in man, it contains follicles enriched with FS cells, mainly at its perimeter (i.e., the marginal zone), likely functioning as a subpopulation of pituitary stem cells (8). The pars tuberalis is well defined in most mammalian species, including man, and surrounds the infundibular stem (9). The floor of the sella, or lamina dura, abuts the sphenoid sinus, allowing direct surgical access to the pituitary by the transsphenoidal route. Other important boundaries to the pituitary gland are the cavernous sinus laterally, which contain the internal carotid artery surrounded with sympathetic fibers, and the cranial nerves III, IV, V (ophthalmic and maxillary branches), and VI (Fig. 7). The optic chiasm is located superiorly, separated from the pituitary by the cerebrospinal fluid-filled suprasellar cistern and the dural roof of the pituitary, the diaphragma sella.
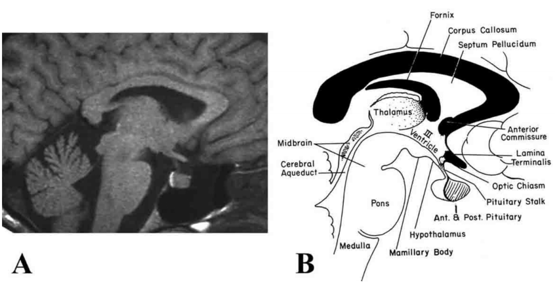
Figure 6. (A) Magnetic resonance image (MRI) and (B) corresponding schematic illustration of the human hypothalamus (H) and pituitary gland seen in sagittal orientation. Note the high intensity or "bright spot" of the posterior pituitary by MRI in (A), sharply defining the boundary between the anterior pituitary gland. III = third ventricle (Modified from Lechan RM. Neuroendocrinology of Pituitary Hormone Regulation. Endocrinology and Metabolism Clinics 16:475-501, 1987.)
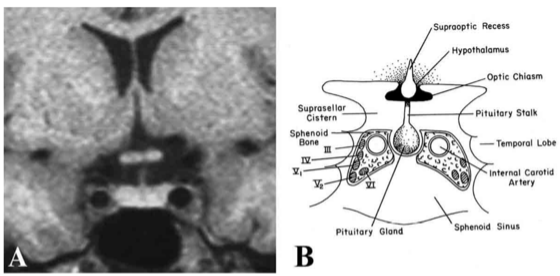
Figure 7. (A) MRI and (B) schematic image of the pituitary fossa and its anatomic relationships seen in coronal orientation. The cavernous sinus contains the internal carotid artery and cranial nerves III, IV, V1, V2, and VI. The optic chiasm resides immediately above the pituitary gland and is separated from it by a cerebrospinal fluid-filled cistern. (Modified from Lechan RM. Neuroendocrinology of Pituitary Hormone Regulation. Endocrinology and Metabolism Clinics 16:475-501, 1987.)
Embryologic Anatomy
The posterior lobe of the pituitary gland is smaller than the anterior lobe and embryologically derives from the neural primordia as an outpouching from the floor of the third ventricle. As a direct, anatomic extension of the central nervous system, it is not surprising that the posterior pituitary is composed primarily of unmyelinated axons and axon terminals as well as specialized glial cells called pituicytes.
In contrast to the posterior pituitary, the anterior pituitary derives from the oral ectoderm as Rathke's pouch, first seen by the third week of pregnancy in man, and gives rise to both the pars distalis and tuberalis. There is little if any direct nervous innervation to the pars distalis, but cell to cell contact with the neuroectoderm of the primordium of the ventral hypothalamus is critical for differentiation of the anterior pituitary into the five major cell types. This occurs as a result of the release of specific growth and transcription factors such as bone morphogenic protein (BMP)-4 and fibroblast growth factor (FGF)-8 (10). Among the numerous transcription factors involved in positional determination and terminal differentiation of pituitary cell types (Fig. 8), the Notch signaling pathway serves a major role in mediating epigenetic regulation of lineage commitment through activation of non-coding RNAs and chromatin-histone interactions (11,12). Recent evidence has also indicated a key role for SOX 2 and SOX3 in regulating pituitary morphogenesis both in rodent and man (13). In humans, mutations of early transcription factors like Rpx, Prop-1 and Pit-1 lead to variable degrees of pituitary insufficiency (10). Once the pituitary matures, the ability of the hypothalamus to communicate with the pars distalis is dependent upon the hypophysial portal system, a vascular link that connects the base of the hypothalamus to the pituitary gland.
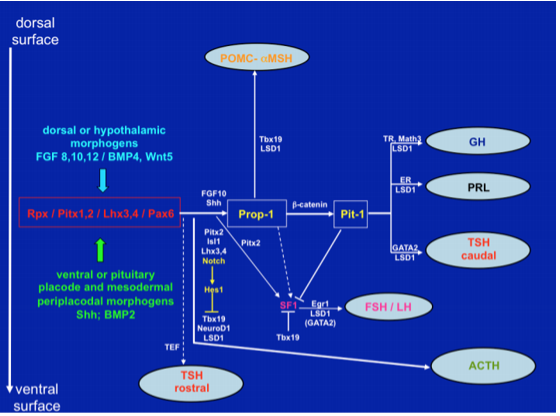
Figure 8. Signaling molecules and transcription factors involved in the development of the mouse anterior pituitary from Rathke’s pouch. In the anterior lobe somatotrophs, lactotrophs and caudally-placed thyrotrophs derive from a common lineage, determined by Prop-1 and Pit-1. Independent lineages are observed for a rostrally-placed group of thyrotrophs, corticotrophs, gonadotrophs and intermediate lobe melanotrophs. All cell types are committed to a specific lineage through activation of Notch signaling at the placodal stage. (Adapted from Cohen and Radovick, Endocrine Reviews 23: 431-442, 2002; Zhu X, Gleiberman AS, Rosenfeld MG, Physiol Rev 87: 933-963, 2007; Zhu X, Wang J, Ju B-G, Rosenfeld MG, Curr Op Cell Biol 19: 605-611, 2007).
Microscopic Anatomy
Microscopically, the anterior pituitary is composed of nests or cords of cuboidal cells organized near venous sinusoids lined with a fenestrated epithelium into which secretory products from the anterior pituitary are collected. Classically, five cell types and six secretory products of the anterior pituitary gland can be identified immunocytochemically including the somatotrophs (growth hormone), lactotrophs (prolactin), corticotrophs (adrenocorticotropic hormone), thyrotropes (thyroid-stimulating hormone), and gonadotrophs (luteinizing hormone and follicle-stimulating hormone) (14). It is well recognized, however, that the anterior pituitary is vastly more complicated. In addition to morphological and physiological evidence for heterogeneity among the classical anterior pituitary cell types (15-18) and the presence of clusters of a unique cell type, the folliculo-stellate cell (19), the anterior pituitary can also synthesize numerous other nonclassical peptides, growth factors, cytokines, binding proteins and neurotransmitters listed in Table 2 that are important for paracrine and/or autocrine control of anterior pituitary secretion and/or cell proliferation under defined physiological conditions (20). Pituitary stem cells have now been recognized in adult mammalian pituitaries as a group of Notch-, Shh-, Wnt- and Hes1-positive elements without hormonal production, primarily residing in the marginal zone around the pituitary cleft (21). However, it is possible that more than a single stem cell type is present in the anterior pituitary. In fact, in rodents, a number of cell groups with stemness potential have been identified, including a subpopulation of folliculostellate cells having the ability to form cell colonies in vitro, a heterogeneous SOX2-positive, SOX9-negative, sphere-forming cell population, a Nestin-positive, potentially adult, progenitor group, and GFRa2-positive (Glial cell line-derived neurotrophic Factor Receptor), sphere-forming cells with clear features of multipotent elements (22). GFRa2-positive cells have also been observed in the marginal zone of the adult, human pituitary (23).
Table 2. Nonclassical Anterior Pituitary Substances and Cell(s) of Origin
|
Substances
|
Cell Types
|
PEPTIDES
|
ACTIVIN B, INHIBIN, FOLLISTATIN
|
F, G
|
ALDOSTERONE STIMULATING FACTOR
|
UN
|
ANGIOTENSIN II (ANGIOTENSINOGEN, ANGIOTENSIN I
|
--
|
CONVERTING ENZYME, CATHEPSIN B, RENIN)
|
C, G, L, S
|
ATRIAL NATURETIC PEPTIDE
|
G
|
CORTICOTROPIN-RELEASING HORMONE-BINDING PROTEIN
|
C
|
DYNORPHIN
|
G
|
GALANIN
|
L, S, T
|
GAWK (CHROMOGRANIN B)
|
G
|
GROWTH HORMONE RELEASING HORMONE
|
UN
|
HISTIDYL PROLINE DIKETOPIPERAZINE
|
UN
|
MOTILIN
|
S
|
NEUROMEDIN B
|
T
|
NEUROMEDIN U
|
C
|
NEUROPEPTIDE Y
|
T
|
NEUROTENSIN
|
UN
|
PROTEIN 7B2
|
G, T
|
SOMATOSTATIN 28
|
UN
|
SUBSTANCE P (SUBSTANCE K)
|
G, L, T
|
THYROTROPIN RELEASING HORMONE
|
G, L, S, T
|
VASOACTIVE INTESTINAL POLTPEPTIDE
|
G, L, T
|
GROWTH FACTORS
|
BASIC FIBROBLAST GROWTH FACTOR
|
C, F
|
CHONDROCYTE GROWTH FACTOR
|
UN
|
EPIDERMAL GROWTH FACTOR
|
G, T
|
INSULIN-LIKE GROWTH FACTOR I
|
S, F
|
NERVE GROWTH FACTOR
|
UN
|
PITUITARY CYTOTROPIC FACTOR
|
UN
|
TRANSFORMING GROWTH FACTOR ALPHA
|
L, S, G
|
VASCULAR ENDOTHELIAL GROWTH FACTOR
|
F
|
CYTOKINES
|
INTERLEUKIN-1 BETA
|
T
|
INTERLEUKIN-6
|
F
|
LEUKEMIA INHIBITORY FACTOR
|
C, F
|
NEUROTRANSMITTERS
|
ACETYLCHOLINE
|
C, L
|
NITRIC OXIDE
|
F
|
C = corticotroph, F = folliculostellate cell, G = gonadotroph, L = lactotroph,
S = somatotroph, T = thyrotroph, UN = unknown
Blood Supply
The pars distalis of the anterior pituitary gland receives little or no arterial blood supply from branches of the internal carotid artery (24,25), while the posterior pituitary is fed by an anastomotic arterial circle derived from each of the inferior hypophysial arteries as they pierce the cavernous sinus (Fig. 9). Rather, the pars distalis is supplied by venous blood delivered through the long portal veins that descend along the ventral surface of the pituitary stalk and interconnect capillary beds in the pars distalis with specialized capillary beds of the portal capillary system in the base of the hypothalamus called the median eminence (Fig. 9). In turn, the portal capillary plexus in the median eminence receives arterial blood from a separate branch of the internal carotid artery, the superior hypophysial artery, after the internal carotid artery ascends from the cavernous sinus. In addition to venous blood draining from the hypothalamus, the pars distalis also receives venous blood draining from the posterior pituitary through the short portal vessels, giving rise to approximately 30 per cent of the total blood supply to the anterior pituitary (26,27). The perfusion sequence of arterial blood first reaching the posterior pituitary and the median eminence, followed by venous drainage to the anterior pituitary can visualized in man using rapidly enhanced magnetic resonance images (dynamic MRI) (28) (Fig. 10). As a result of the venous blood flow pattern to the pituitary, the pars distalis is in a unique position where it can receive humeral information from the hypothalamus and the posterior pituitary, as well as substances circulating in the peripheral bloodstream. Due to the location of pars tuberalis cells in the pituitary stalk and ventral surface of the median eminence, adjacent to the portal capillary plexus, it is likely that these cells also contribute to the humeral substances that are carried by a vascular route to the pars distalis (29), although its physiological significance is unknown.
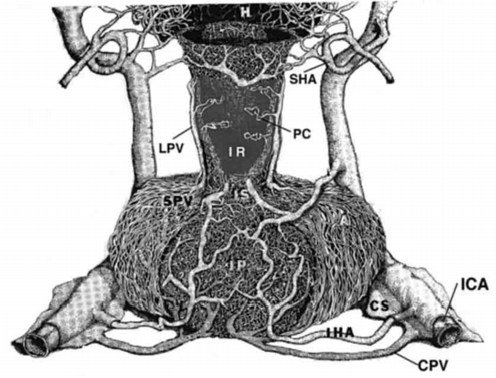
Figure 9. Drawing of the vasculature of the primate anterior and posterior pituitary gland. A portion of the pituitary stalk (I) has been cut away to visualize the infundibular recess (IR) and portal capillaries (PC). CPV = confluent pituitary veins, CS = cavernous sinus, H = hypothalamus, IC = internal carotid artery, IHA = inferior hypophysial artery, IP = infundibular processes or posterior pituitary, LPV = long portal veins, SHA = superior hypophysial artery, SPV = short portal veins. (From Lechan RM, Functional Microanatomy of the Hypophysial-Pituitary Axis, in Melmed, S (Ed), Oncogenesis and Molecular Biology of Pituitary Tumors, Frontiers of Hormone Research, 20: 2-40, 1996.)
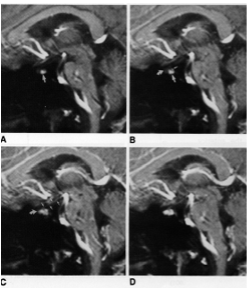
Figure 10. (A-D) MRI of sequential sequences of the stalk and pituitary gland in sagittal orientation following the intravenous administration of gadolinium. (A) Appearance prior to gadolinium. (B) Following gadolinium, the posterior pituitary is the first structure to show contrast enhancement. (C) This is followed by the pituitary stalk (arrow) and then finally (D) the anterior pituitary. (From Yuh et al, AJNR 15: 101-108, 1994.)
Venous drainage from the anterior pituitary to the systemic circulation is through adenohypophysial veins located at a sulcus separating the anterior pituitary from the posterior pituitary (24). Other than the short portal vessels, venous drainage from the posterior pituitary collects into neurohypophysial veins, which together with adenohypophysial veins, extend as common vessels (confluent pituitary veins) to the cavernous sinus (Fig. 9). The cavernous sinus is enriched by an additional draining system composed of paravascular spaces around the hypophysial arteries and veins, variably interconnected with intradural channels lined by a lymphatic endothelium, giving rise to the pituitary glymphatic system (30). It provides a route for transport and clearance of intercellular liquids and metabolites into the blood circulation, allowing for volume transmission of soluble information at the level of the hypothalamic-pituitary unit (for anatomical details of this circuitry, see the section on the Glymphatic system and sinus-associated dural glymphatics).
ANATOMY OF THE HYPOTHALAMUS
Gross Anatomy
The hypothalamus lies directly above the pituitary gland (Fig. 11) and occupies approximately 2 per cent of the brain volume. It is composed of a number of cell groups (Fig. 12) as well as fiber tracts that are symmetric about the third ventricle. In sagittal section, the hypothalamus extends from the optic chiasm, lamina terminalis and anterior commissure rostrally to the cerebral peduncle and interpeduncular fossa caudally (Fig. 11). The cavity of the third ventricle lies in the midline. In coronal section (Fig. 13), each of the two symmetric walls of the hypothalamus can be divided into four surfaces: a lateral surface contiguous with the thalamus, subthalamus and internal capsule, the latter dividing the hypothalamus from the corpus striatum; a medial surface extending to the wall of the third ventricle, covered by ependymal cells; a superior surface corresponding to the hypothalamic sulcus that separates the hypothalamus from the central mass of the thalamus; and an inferior surface that is in continuity with the floor of the third ventricle. The external surface of the hypothalamic floor (Fig. 14) gives rise to a median protuberance called the tuber cinereum (or gray swelling due to the pale bluish color of the blood vessels seen in the postmortem human brain), whose central part extends anteriorly and downward into a funnel-like process, the infundibulum or median eminence. The infundibulum is in direct continuity with the infundibular stem of the posterior pituitary gland, and together with the pars tuberalis of the anterior pituitary, forms the pituitary stalk (Fig. 6). Two additional symmetric eminences, the lateral eminences, corresponding to the most lateral portion of the hypothalamic wall and the postinfundibular eminence, as well as the symmetric mammillary bodies, complete the macroscopic morphology of the hypothalamic floor.
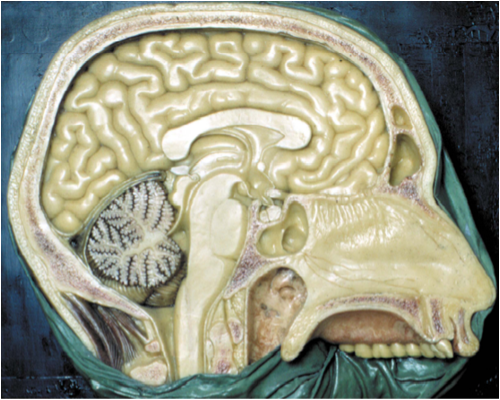
Figure 11. Midsagittal section of the human brain (from the XIX century wax collection of human brains at the Museum of the Department of Human Anatomy of the University of Bologna, Italy). The hypothalamus (asterisk) lies above the pituitary gland (cross) and has as its boundaries (1) the anterior commissure and lamina terminalis anteriorly; (2) mammillary bodies and midbrain posteriorly, and (3) thalamus superiorly. (From Lechan R.M. and Toni R., Regulation of Pituitary Function, in Korenman S.G (Ed), Atlas of Clinical Endocrinology, Current Medicine, vol IV, 1-25, 2000).
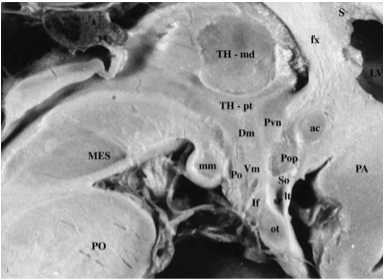
Figure 12. Magnified view of a fixed human brain in midsagittal orientation. The third ventricle makes up the core of the hypothalamus and extends into the pituitary (or infundibular) stalk, creating the infundibular recess. Many of the major cell groups are located near the midline. These include (from rostral to caudal) the preoptic nucleus (Pop), paraventricular nucleus (Pvn), dorsomedial nucleus (Dm), ventromedial nucleus (Vm), arcuate (or infundibular) nucleus (If), posterior hypothalamic nucleus (Po), and medial mammillary nucleus (mm). Ac = anterior commissure, fx = fornix, lt= lamina terminalis, ot = optic tract and chiasm, Lv = lateral ventricle, MB = midbrain, PN = pons, Sr = supraoptic recess, T = thalamus. (From Lechan R.M. and Toni R., Regulation of Pituitary Function, in Korenman S.G (Ed), Atlas of Clinical Endocrinology, Current Medicine, vol IV, 1-25, 2000).
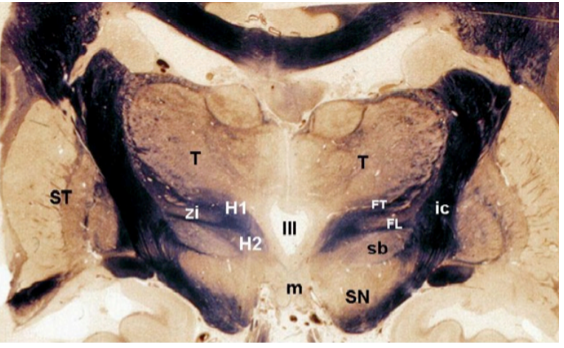
Figure 13. Coronal section of a fixed human brain at the level of the posterior hypothalamus. The third ventricle (III) lies in the midline directly above the mammillary bodies (m). The subthalamus (sb), zona incerta (zi) and thalamus (T) are located at the superior border of the hypothalamus, whereas the corpus striatum (ST) is located laterally. FL = fasciculus lenticularis, FT = fasciculus thalamicus, ic = internal capsule, SN = substantia nigra, H1 = field H1 of Forel; H2 = field H2 of Forel. (From Toni R, Malaguti A, Benfenati F, Martini L: The human hypothalamus: a morphofunctional perspective. J Endocrinol Invest 27 (supp to n.6), 73-94, 2004.)
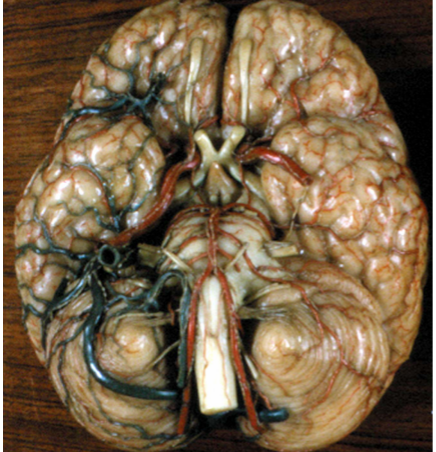
Figure 14. Basal view of the brain showing the external surface of the floor of the hypothalamus and its arterial vessels. The infundibulum (I) lies posteriorly to the optic tracts and chiasm (ot) and anterior to the mammillary bodies (m). The arterial circle of Willis surrounds the hypothalamic floor and provides the arterial supply to the hypothalamic nuclei and fiber tracts. ac = anterior cerebral artery, aco = anterior communicating artery, b = basilar artery, ic = internal carotid artery, P = pons, pc = posterior cerebral artery, pco = posterior communicating artery. (From the XIX century wax collection of human brains at the Museum of the Department of Human Anatomy of the University of Bologna, Italy.)
Embryologic Anatomy
The diencephalon derives from the caudal part the pro-encephalic vesicle, which is the cranial expansion of the primitive neural tube, and the hypothalamus develops from the lateral wall of the diencephalon by extending ventrally to a groove called the “hypothalamic sulcus” that appears early in the lateral wall of the diencephalon (Figure 15). Therefore, the hypothalamus can be considered a ventral derivative of the neural tube and to originate from the embryonic basal plate (31). Since the basal plate is the source of all skeletal and autonomic motor neurons in the CNS, by inference, the hypothalamus has also been considered a motor system (32). Indeed, neuroendocrine neurons that are involved in the regulation of the anterior and posterior pituitary secretion clearly have secretomotor functions. However, some authorities believe that the basal (motor) plate of the neural tube ends at the level of the mesencephalon, and that the diencephalon (hypothalamus included), is actually a derivative of the dorsal or alar plate, which is primarily sensory (33). Partial confirmation of this idea has been recently provided by the evidence that mouse embryonic stem cells may spontaneously differentiate into neurons expressing the Rax gene, a marker common to both the preoptic / tuberal hypothalamus and neural retina (a sensory structure), but do not express the Irx3 and En2 genes, typical of the midbrain structures (34). These findings are consistent with the presence of neurosecretory cells with sensory properties in the forebrain of invertebrates and fish (35), suggesting evolutionarily conserved sensory properties of neuroendocrine hypothalamic cells.
Within the neural tube, dividing hypothalamic neuroblasts remain confined within the cell layer adjacent to the ependymal canal (ependymal or ventricular layer), whereas postmitotic elements migrate more laterally into a cell-dense region (mantle layer) before reaching their final destination (36) (Figure 16). Collectively, hypothalamic progenitors reach their final patterning and location in ventral and dorsal regions by exposure to two, specific, transcription factors, the ‘ventralizing’ Shh and subsequently the ‘dorsalising’ Bmp7. As a result, specific transcription factor codes are established, leading to early differentiation of ventrolateral progenitors expressing the homeobox gene product, Pax 7 (37). Outgrowth of neural process occurs at the most lateral borders of the hypothalamic mantle layer to give rise to tangential fiber tracts that course parallel to the ependymal canal and connect hypothalamic neurons with cranial and caudal portions of the developing neural tube. These fiber tracts are highly ordered into spatial and temporal patterns (38). Early connections include those with the midbrain (mammilotegmental tract) and hippocampus (stria terminalis), followed by those with the thalamus (mammilothalamic tract) (39).
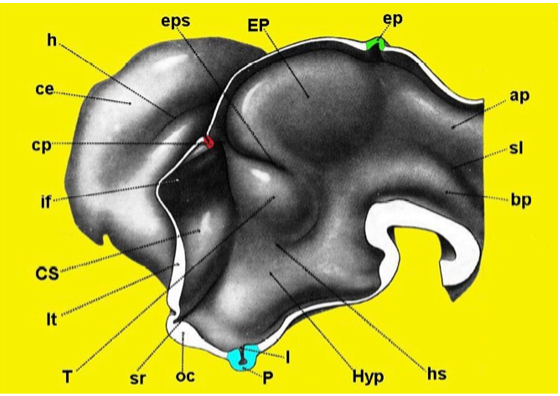
Figure 15. Three-dimensional reconstruction of the developing proencephalon in the human embryo. Note that at the level of the inferior portion of the lateral wall is the region of the hypothalamus (Hyp) with the infundibular bud (I) and pituitary anlage (P) (Redrawn from Hines M, J Comp Neurol 34: 73-171,1922.) ap = alar plate, bp = basal plate, ce = cerebral hemisphere, cp = choroidal plexus, CS = corpus striatum, ep = epiphysis, EP = epithalamus, eps = epithalamic sulcus, h = hippocampal fissure, hs = hypothalamic sulcus, if = interventricular foramen, lt = lamina terminalis, oc = optic chiasm, sl = sulcus limitans, sr = supraoptic recess, T = thalamus.
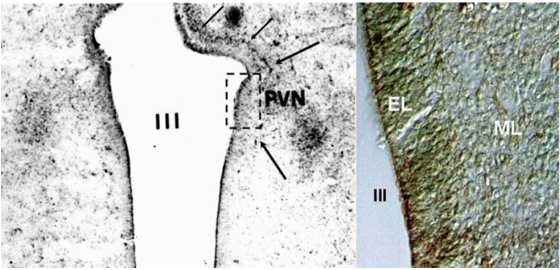
Figure 16. Coronal section of the anterior hypothalamus in a human fetus of gestational age 12-14 weeks, counterstained with methylgreen and thionine. (A) Note that from the wall of the third ventricle, constituting the ependymal layer of the neural tube, a front of developing cells (arrows) migrate laterally towards the mantle layer to give rise to the primordium of the paraventricular nucleus (PVN). (B) High magnification of the image included in the rectangle shown in A. Note the high cellular density in the ependymal layer (EL) of the neural tube contrasts with the more diffuse distribution of migrating neuroblasts in the developing mantle layer (ML). III = third ventricle.
Organization of the hypothalamus into specific nuclear groups occurs in a temporal and spatial pattern both in rodents (38,39) and man (40), such that the entire preoptic to posterior lateral hypothalamus followed by the medially-located, neurohypophysial centers and the main part of the medial preoptic and tuberal hypothalamus all arise during an early phase of development, whereas the periventricular hypothalamus, the floor of the third ventricle and mammillary complex develop later (see Section C, Microscopic Anatomy). Peak birth dates of specific hypothalamic nuclei in the primate are shown in Table 3.
Table 3. Birthdates of Hypothalamic Nuclei in the Primate Brain
|
Hypothalamic nucleus
|
Peak birthdate
|
MPA
|
e43-e45
|
SCN
|
e30-e43
|
SON
|
e30-e38
|
PVN
|
e40-e43
|
ARC
|
e30
|
VMN
|
e30
|
DMN
|
e38
|
(Based on van Eerdenburg FJCM, Rakic P. Early neurogenesis in the anterior hypothalamus of the rhesus monkey. Dev. Bran Res. 79: 290-296, 1994)
In addition to generalizations above regarding the development of specific hypothalamic nuclei, there are developmental differences that distinguish neuroendocrine neurons in the hypothalamus from non-neuroendocrine neurons. Namely, neuroendocrine neurons, including those that give rise to the tuberoinfundibular and magnocellular neurohypophysial systems that are involved in regulation of the anterior and posterior pituitary, respectively (see later), differentiate immediately after closure of the neural tube, even before reaching their final destination within hypothalamic nuclei (41). This phenomenon has been clearly demonstrated for GnRH neurons, that are fully differentiated at the level of the olfactory placode, even before migrating into the preoptic region of the hypothalamus (42). Similarly, neuroblasts immunoreactive for the hypophysiotropic peptides, somatostatin and thyrotropin-releasing hormone, can be identified in the human fetal hypothalamus at the interface between the ependymal and mantle layers during a developmental stage that precedes complete formation of the PVN (43,44).
A number of genes have now been identified that regulate the temporal and spatial patterns of differentiation of hypothalamic cell groups. The POU III-related homeobox genes, Brn-1, Brn2, and Brn4, are involved in the development of the periventricular and medial parts of the hypothalamus (45). Transgenic mice with loss of function mutations or with targeted disruption of the Brn-2 gene, lack both the PVN and supraoptic nuclei, and have no somatostatin-producing neurons in the periventricular hypothalamus (46,47). Expression of Brn-2 is dependent upon transcription factors Sim1 and ARNT2, since mutations of these genes in transgenic mice result in a phenotype that is similar to the Brn-2 KO mice (48-50). Similarly, mice with decreased Sim1 expression have reduced vasopressin and oxytocin neurons, and develop a hyperphagic and obese phenotype (51). A number of other genes have been identified that are involved in differentiation of specific hypothalamic nuclei and are listed in Table 4. Temporal and spatial expression of many of these genes is selectively regulated by circulating sex hormones (52) and peripheral satiety signals such as leptin (53), suggesting that innate neuroendocrine behavioral responses are epigenetically influenced during the embryonic and fetal life. Indeed, epigenetic imprinting in the mammalian hypothalamus has been recognized for a number of maternally silenced genes by knockout of the paternally-expressed allele including: a) Magel2, encoding a transcriptional regulator whose disruption leads to neonatal growth retardation, excessive post-weaning weight gain, adiposity, reduced food intake, and disappearance of orexin neurons; b) Ndn, encoding the growth suppressor and anti-apoptotic factor, necdin, whose loss leads to reduction in oxytocin and LHRH neurons; c) Nnat, encoding neuronatin that regulates energy homeostasis for which a single nucleotide polymorphism in man is associated with severe childhood and adult obesity; d) Gnasxl, encoding the transcript of the Gsα isoform, XLαs, whose inactivation results in a hypermetabolic phenotype with decreased adiposity, increased glucose tolerance and insulin sensitivity; and e) Pag3, that encodes a Kruppel-type zinc finger transcription factor whose absence reduces metabolic rate, lowers the core body temperature, increases adiposity, induces leptin resistance, reduces sympathetic activity, and alters the proportion of neuropetide neurons in the periventricular and medial hypothalamic nuclei. Paternally-imprinted genes have also been recognized in the hypothalamus including Gnas that has selective expression in the PVN and whose constitutive knockout leads to Albright hereditary osteodystrophy characterized by severe obesity, lethargy, glucose intolerance and insulin, TSH and PTH resistance (54).
Table 4. Genes and Transcription Factors Involved in the Development of Specific Regions of the Rodent Hypothalamus
|
Gene
|
Nuclear Region
|
Brn-1, Brn-2, Brn-4
|
PVN, SON, PV, POA, MN, PH
|
Dlx1
|
TH
|
Vgll2, SF-1, Sox14, Satb2,
|
VMN
|
Fezf1, Nkx2-2, COUP-TFII
|
|
Gsh1, Mash1
|
ARC, VMN
|
Otp
|
PVN, SON, PV, POA, AH, ARC
|
rPtx-2
|
TH, MN
|
Sim1
|
PVN, SON
|
Fkh5
|
MN
|
Tst-1
|
MN, PH
|
(Based on Markakis E. A. Frontiers in Neuroendocrinology, 23: 257-291, 2002; McNay DE, Pelling M, Claxton S, Guillemont F, Ang S-L, Mol Endocr 20: 1623-1632, 2006; Kurrasch DM, Cheung CC, Lee FY, Tran PV, Hata K, Ingraham HA. J Neurosci 27: 13624-13634, 2007.)
AH = anterior hypothalamus, ARC = arcuate nucleus, MN = mammillary nuclei = posterior hypothalamus, POA = preoptic area, PV = periventricular nucleus, PVN = paraventricular nucleus, SON = supraoptic nuclei, TH = tuberal hypothalamus, VMN = ventromedial nucleus
Microscopic Anatomy
BOUNDARIES AND ORGANIZATION OF NEURONAL CELL GROUPS
Using phylogenetic and cytoarchitectonic criteria (55), a number of nuclear groups and fiber tracts are recognized in the vertebrate hypothalamus. These are organized into three major regions including the lateral, medial and periventricular hypothalamus, each having distinct morphological and functional features. In the human hypothalamus, the anterior column of the fornix that extends rostro-caudally through the substance of the hypothalamus to end in the mammillary bodies, and the mammillo-thalamic tract that projects from the mammillary bodies upward to the thalamus, create an anatomical boundary that divides the hypothalamus into medial and lateral subdivisions (Fig. 17). Contained within the medial subdivision is the periventricular subdivision, a 5-6 cell layer thick nuclear group surrounding the third ventricle that is easily recognized in rodents using standard vital stains, but has less clear anatomical boundaries in the human brain.
Both the medial and periventricular subdivisions of the mammalian hypothalamus contain a high density of neuronal cell bodies organized into nuclear groups (Tables 5 and Fig. 17) and in the human brain, has been classified with a number of different synonyms (Table 6). Both subdivisions are crucial for the regulation of the anterior and posterior pituitary gland. The medial hypothalamus also contains nuclear groups that serve as relay centers for highly differentiated neural information coming from the neocortex, limbic system and autonomic sensory centers in the brainstem involved in initiation phases of specific homeostatic behaviors such as thirst, hunger, thermoregulation, the sleep-wake cycle, and reproductive behavior (55). The lateral hypothalamus occupies the largest portion of the hypothalamus by volume. However, it has relatively fewer neurons compared to the medial hypothalamus, and only a limited number of nuclear groups intercalated within a massive fiber system, the medial forebrain bundle (MFB). It is through this fiber system that information from the medial forebrain (amygdala, hippocampus, septum, olfactory system, neocortex) and the brainstem is carried to the medial and periventricular hypothalamic subdivisions, delegating an important role to the lateral hypothalamus to influence homeostatic control systems elaborated by the medial hypothalamus. Figure 18 schematically depicts major interrelationships between the periventricular, medial and lateral hypothalamic subdivisions and the rest of the brain.
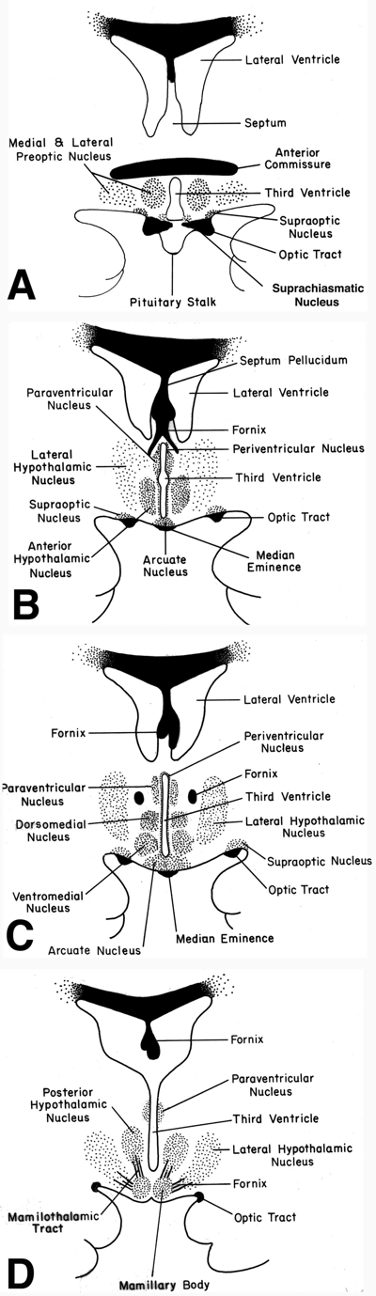
Figure 17. Schematic representation of the human hypothalamus in coronal orientation (A-D: rostral to caudal), demonstrating the location of major nuclear groups. Drawings correspond to MRI images in Fig. 26. Using the fornix (fx) as an anatomic landmark as it passes through the mid-portion of the hypothalamus on each side of the third ventricle, it is convenient to divide the hypothalamus into medial and periventricular zones (that lie largely medial to the fornix) and a lateral zone (that lies lateral to the fornix). The medial and periventricular zones contain most of the hypothalamic cell groups, and the lateral zone contains relatively fewer neurons. This is because the lateral zone is largely composed of a massive bidirectional fiber pathway – the medial forebrain bundle – that extends through the hypothalamus and interconnects it with the limbic system and brainstem autonomic centers.
Table 5. Major Hypothalamic Cell Groups in Mammals
|
PERIVENTRICULAR ZONE
PERIVENTRICULAR NUCLEUS
SUPRACHIASMATIC NUCLEUS
PARAVENTRICULAR NUCLEUS
ARCUATE NUCLEUS
MEDIAL ZONE
MEDIAL PREOPTIC NUCLEUS
ANTERIOR HYPOTHALAMIC NUCLEUS
DORSOMEDIAL NUCLEUS
VENTROMEDIAL NUCLEUS
PREMAMMILLARY NUCLEUS
MAMMILLARY NUCLEUS
POSTERIOR HYPOTHALAMIC NUCLEUS
|
LATERAL ZONE
LATERAL PREOPTIC NUCLEUS
LATERAL HYPOTHALAMIC NUCLEUS
SUPRAOPTIC NUCLEUS
|
rostral to caudal order of appearance in each zone
Based on the anatomical classification of Nauta WJH and Haymaker W, Hypothalamic nuclei and fiber connections.: Haymaker W, Anderson E, Nauta WJH (eds); The Hypothalamus, Charles C Thomas Publisher, 1969, pp 136-209
Table 6. Terminology of hypothalamic nuclei in the human brain (rostral to caudal order of appearance)
|
Spiegel
Zweig
1919
|
Clark
1936
|
Brockhaus
1942
|
Khulenbeck
Heimaker / Nauta
1949-69
|
Feremutsch
1955
|
Diepen
1962
|
Schattelbrand
Wahren
1977
|
Braak
1987
|
Swaab
1985-92
|
GTD
|
POA
|
n. prothal. periventr.
|
nn. lineae medianae
preoptic periventric.n.
|
a. periventr. hypothal.
e / ba
|
preoptic
groups
|
n. prothal. periventr.
d / int / v
|
periventricular n.
|
|
SCN
|
POA
|
n.ovoideus
|
SCN
|
a. periventr. hypothal.
communis
|
SCN
|
n. ovoideus
|
SCN
|
SCN
|
GTD
|
POA
|
n. prothal.
princip.
o / ce / v
|
medial preopt.ic n.
anterior hypothal. n.
periventr. preoptic n.
lateral preoptic n.
|
a. periventr. hypothal anterior
a. lateralis hypothal anterior
a. lateralis hypothal. reticularis
|
lateral
hypothal.
n.
|
n. prothal.
principalis
o / ce / v / l
|
chiasmatic grey
cuneiform n.
uncinate n.
|
POA
OVLT
|
GTD
|
POA
|
nucleus
intermed.
|
medial preopt. n.
anterior hypoth. n.
|
intermediate lateral hypothal. a.
|
|
n. prothal.
princip.
ce / v
|
intemediate n.
|
SDN
|
GTD
|
POA
|
orolateral
hypothal. n.
|
n. supraoptic
diffusum
|
|
|
|
retrochiasmatic
n.
|
|
SON
|
SON
|
SON
|
SON
|
SON
|
SON
|
SON
|
SON*
|
SON
|
PVN
|
PVN
|
PVN
|
PVN
|
PVN
|
PVN
|
PVN
|
PVN*
|
PVN
|
MII
|
|
|
arcuate
or infundibular n.
|
a. periventr. basalis posterior
|
INF
|
INF
|
INF°
|
|
GTD
|
VMN
|
|
VMN
|
a. lateralis hypothal. ventromed.
|
VMN
|
VMN
|
VMN, postero-medial n.
|
|
GTD
|
DMN
|
|
DMN
|
a. periventr. hypothal. communis
a. lateralis hypothal. posterior
|
DMN
|
DMN
|
DMN
|
|
GTD
|
LHA
|
|
TMN
|
mammillo-infundibular n.
|
TMN
|
TMN
|
TMN
|
|
|
PN
|
|
PN
|
|
|
|
PN
|
|
PFN
|
|
|
PFN
|
a. lateralis hypothal. posterior (parafornicalis)
|
PFN
|
PFN
|
|
|
GTD
|
|
|
DN
|
n. paraventricularis pars caudalis
a. lateralis hypothal. posterior
pars dorsalis
|
a. dorsalis
|
n. dorsalis
|
|
|
GTD
|
LHA
|
|
LHA
|
a. lateralis hypothal. reticularis
pars principalis
|
pars lateralis tubero-mammillaris
|
n. lateralis
|
|
|
nn. tuberis
|
nn
tuberis
|
|
nn. tuberis laterales
|
n tuberis lateralis hypothalami
|
nn. tuberis
lateralis
|
n. tuberis
lateralis
|
LTN
|
LTN
|
|
MMN
|
|
MMN
|
n. corporis mammillaris
|
|
MMN
|
|
|
|
LMN
|
|
LMN
|
|
|
LMN
|
|
|
|
n. interc.
|
|
n. interc.
|
n. interc.hypothal.
|
|
n. interc.
|
|
|
DMN dorsomedial nucleus, GTD = griseum tuberis diffusum, INF = infundibular nucleus, LHA = lateral hypothalamic area, LMN = lateral mammillary nucleus, LTN = lateral tuberal nucleus, MII = massa infundibularis intermedia, MMN = medial mammillary nucleus, OVLT = organum vasculosum lamina terminalis, PFN = perifornical nucleus, PN = posterior nucleus, POA = preoptic area, PVN = paraventricular nucleus, SCN = suprachiasmatic nucleus, SDN = sexual dimorphic nucleus. SON = supraoptic nucleus, TMN = tuberomammillary nucleus, VMN = ventromedial nucleus, a. = anterior; ce = centralis, d = dorsalis, int. = intermedius, l = lateralis, n. interc= nucleus intercalatus, nucleus intermed. = nucleus intermedius, n. prothal. periventr. = nucleus prothalamicus periventricularis, n. prothal. princip. = nucleus prothalamicus principalis, o = oralis, v = ventralis; * = associated with surrounding accessory magnocellular neurosecretory nuclei; ° = including cranially the periventricular nucleus. Based on Toni R, Malaguti A, Benfenati F, Martini L: The human hypothalamus: a morphofunctional perspective. J Endocrinol Invest 27, supp to n.6, 73-94, 2004
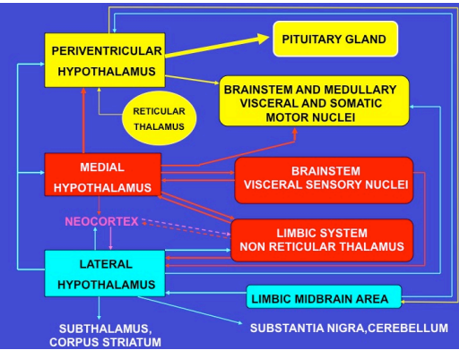
Figure 18. Schematic representation of the major neural pathways connecting the periventricular, medial and lateral hypothalamic subdivisions with the rest of the brain. Groups with identical colors are functionally linked.
Each of the three hypothalamic subdivisions can be further divided along the rostral-caudal axis into the: a) anterior or chiasmatic region, extending between the lamina terminalis and the anterior limit of the infundibular recess; b) median or tuberal region, extending between the infudibular recess and the surface of the anterior column of the fornix; and c) posterior or mammillary region, extending between the anterior column of the fornix and the caudal limit of the mammillary bodies.
Recent tract-tracing and morphofunctional studies in rodents have proposed a functional perspective of hypothalamic subdivisions aimed at coordinating behavioral responses like feeding, reproduction and defense/exploration with autonomic and neuroendocrine responses. In particular, it has been suggested that specific nuclei in the rostral part (chiasmatic region) of the periventricular subdivision (namely the preoptic area) and dorsal zone of the tuberal region (namely the dorsomedial nucleus) reciprocally interact to provide outputs unique for different pools of neuroendocrine neurons located along the walls of the third ventricle (collectively considered as a periventricular motor zone), coupled to outputs to selective pools of autonomic neurons in all hypothalamic subdivisions (collectively considered as preautonomic cell groups). This neuronal network would be responsible for constant, reproducible but different patterns of endocrine and autonomic activation in response to specific homeostatic signals (hunger, sexual desire, motivated motor activity), constituting a hypothalamic visceromotor pattern generator (HVPG). In this manner, it would be clearly recognized that the HVPG is comprised of a contingent of neurons interposed between the classical periventricular and medial hypothalamic subdivisions (56).
CIRUMVENTRICULAR ORGANS
One of the most important regions in the hypothalamus that is essential for regulation of the pituitary gland is the median eminence, a midline structure located in the basal hypothalamus ventral to the third ventricle and adjacent to the arcuate nucleus. It is here that all hypophysiotropic hormones converge before they are conveyed to the pituitary gland. The median eminence is one of seven so called circumventricular organs situated as midline structures in the walls of the lateral, third or fourth ventricles (57,58). Other circumventricular organs include the organum vasculosum of the lamina terminalis, subfornical organ, choroid plexus, pineal gland, subcommissural organ and area postrema(Fig. 19). Characteristically the circumventricular organs contain a rich capillary plexus and with the exception of the subcommissural organ, have a fenestrated endothelium rendering the structures outside of the blood brain barrier. This morphologic feature together with the presence of neural elements contacting the fenestrated capillaries allows the secretion of brain-derived products into the peripheral circulation and/or makes circumventricular organs targets for blood-born information which can then be transmitted to the brain (59).
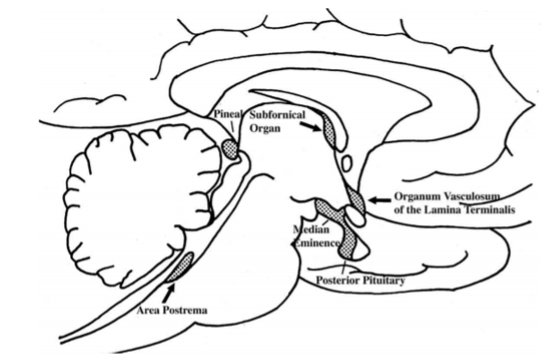
Figure 19. Location of circumventricular organs in the rat brain. AP = area postrema, ME = median eminence, OVLT = organum vasculosum of the lamina terminalis, P = pineal gland, PP = posterior pituitary, SFO = subfornical organ. (Modified from Saper and Breder, New England Journal of Medicine 330: 1080-1886, 1994.)
The median eminence is a highly organized structure containing three zones: the ependymal zone, the internal zone (or zona interna) and the external zone (or zona externa) (60,61) (Fig. 20A). The ependymal zone forms the floor of the third ventricle and has some very specialized features including densely formed tight junctions between adjacent cells and highly specialized cells, tanycytes, that extend bleb-like protrusions and microvilli into the cerebrospinal fluid (CSF) at their ventricular surface and long cytoplasmic processes ventrally into the substance of the median eminence (61,62). Since the portal capillaries in the median eminence lie outside of the blood brain barrier, one of the functions of the ependymal zone is to create a barrier to the brain, preventing substances released into the periportal capillary spaces from entering the cerebrospinal fluid (63,64). Tight junctions also can be found at the dorso-lateral margins of the median eminence adjacent to the neuropil of the arcuate nucleus created by perivascular tanycyte processes (48), thereby compartmentalizing all substances entering the periportal capillary spaces within the confines of the median eminence, itself. Norsted et al (49), however, have demonstrated the absence of endothelial barrier antigen in blood vessels at the far ventro-medial aspect of the arcuate nucleus close to the border between the walls and floor of the third ventricle, and propose the lack of a blood brain barrier in this region. Allowing blood-born substances including gut peptides, glucose, amino acids and fatty acids to enter the arcuate nucleus in this region may be an important homeostatic mechanism that contributes to the regulation of appetite and satiety (see later).
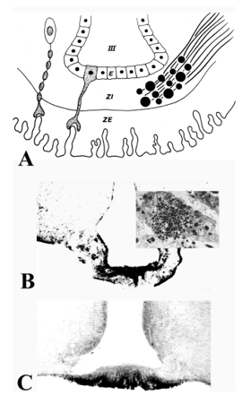
Figure 20. (A) Schematic diagram of the median eminence showing the organization of its three major zones: ependymal zone (E), internal zone (ZI), and external zone (ZE). ZE is invigilated by portal capillaries which are contacted by axon terminals of the tuberoinfundibular system and by processes of specialized ependymal cells, the tanycytes. (B) Fibers coursing through the ZI are seen immunocytochemically in the rat using antiserum to vasopressin. (C) Fibers terminating in the ZE in close association to portal capillaries (PC) are seen immunocytochemically in the rat using a proTRH-directed antiserum. III = third ventricle. (From Lechan RM, Functional Microanatomy of the Hypophysial-Pituitary Axis, in Melmed, S (Ed), Oncogenesis and Molecular Biology of Pituitary Tumors, Frontiers of Hormone Research, 20: 2-40, 1996.)
Based on their location, morphology, cytochemistry and ultrastructure, tanycytes can be divided into several subtypes including alpha, beta and recently characterized gamma subtypes (65,66). The alpha subtypes line the ventrolateral walls of the third ventricle and beta subtypes line the floor and lateral extensions of the third ventricle. Gamma subtypes are small tanycytes that can be found throughout the substance of the median eminence (67). While presumed to be barrier cells, they likely have other important, neuroendocrine functions that may supersede their role as barrier cells. The close association of tanycyte foot processes with the basal lamina of the portal capillaries and with individual axon terminals (Fig. 21) could create a retractable barrier to regulate the diffusion of secretory products entering or exiting specific regions of the portal capillary plexus or from axon terminals (68,69). This mechanism has been shown to have an important role in the regulation of gonadal function in photoperiod sensitive animals, in which retraction of the tanycyte foot processes from portal vessels during long days allow activation of reproductive function (70). A similar dynamic interaction between glial cells and secretory nerve endings in the posterior pituitary have been described by Beagley and Hatton (71). In addition, the absorption of substances from the CSF at its apical surface for transport to the portal capillaries (62,72,73) could result in a mechanism whereby secretory products released into the CSF have access to the anterior pituitary. Tanycytes may also serve as a scaffolding for axons entering the median eminence during embryologic development, guiding them to their ultimate destination in the external zone (74). Tanycytes express one of the highest concentrations in the brain of type 2 deiodinase (D2) (75), the enzyme responsible for the conversion of thyroxine (T4) into its more biologically potent product, triiodothyronine (T3), the D2 degrading and reactivating enzymes, WSB-1 and VUD-1 (76), thyroid hormone transport (78.). These observations among others are in keeping with recent reports on the important role of tanycytes in control of the hypothalamic-pituitary-thyroid axis and regulating tissue levels of thyroid hormone in the hypothalamus (79-81).
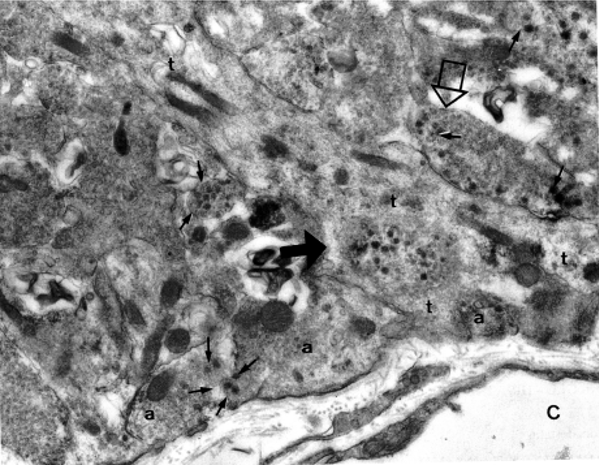
Figure 21. Electron micrograph of the external zone of the median eminence showing the presence of axon terminals (a) and a tanycyte process (t) adjacent to a fenestrated capillary (C) of the portal plexus. One axon (closed arrowhead) has been engulfed by the tanycyte and another (open arrowhead) is separated from the portal capillary space by the tanycyte foot process. Note presence of dense core vesicles (arrows) as well as smaller secretory vesicles in several axon terminals. (From Lechan RM, Functional Microanatomy of the Hypophysial-Pituitary Axis, in Melmed, S (Ed), Oncogenesis and Molecular Biology of Pituitary Tumors, Frontiers of Hormone Research, 20: 2-40, 1996.)
Tanycytes also express a number of embryotic genes (82), suggesting that they may serve as stem cells. Indeed, any damage to tanycytes is repaired by rapid regeneration of these cells to reline the third ventricle (83). Along these lines, tanycytes have been observed to express POMC mRNA (84), raising the possibility that they may have the ability to differentiate into neurons and contribute to the neuronal population in the adjacent arcuate nucleus. Other evidence for tanycyte differentiation into neurons has also been given (82,85). Finally, tanycytes have properties of inflammatory cells and may be capable of producing cytokines and chemokines that contribute to the mechanism of hypothalamic inflammation associated with a high fat intake (personal observations).
The internal zone of the median eminence lies directly below the ependymal zone and is primarily composed of unmyelinated axons of passage of the hypothalamic-neurohypophysial system en route to the posterior pituitary (Fig. 20B). Characteristic of these axons are dilatations or Herring bodies, in which collect large numbers of neurosecretory granules measuring 200 to 350 nm in diameter (Inset, Fig. 20B). The internal zone also contains cytoplasmic processes of tanycytes and axons of passage of the hypothalamic tuberoinfundibular system as they descend into the external zone.
The external zone underlies the internal zone and in addition to the portal capillaries and cytoplasmic extensions of the tanycytes described above, it contains numerous fine calibers, unmyelinated axons and axon terminals of the hypothalamic tuberoinfundibular system (Fig. 20C). Characteristic of these axon terminals are dense-core vesicles ranging from 50 to 130 nm in diameter (Fig. 21). The close proximity of many of the axon terminals to the portal system suggests that these axons are capable of secreting the material stored in their vesicles into the pericapillary spaces and by percolating through the fenestrated endothelium of the portal capillaries, reach the anterior pituitary by way of the long portal vessels. These substances, commonly referred to as hypothalamic releasing and inhibitory hormones on the basis of their ability to stimulate or inhibit anterior pituitary hormone secretion respectively, have been chemically identified and are listed in Table 7.
Table 7. Classic Hypothalamic Releasing and Inhibitory Substances
|
Substance
|
Acids
|
CORTICOTROPIN-RELEASING HORMONE (CRH)
SER - GLU - GLU - PRO - PRO - ILE - SER - LEU - ASP - LEU - THR - PHE - HIS - LEU - LEU-ARG - GLU - VAL - LEU - GLU - MET - ALA - ARG - ALA - GLU - GLN - LEU - ALA - GLN -GLN - ALA - HIS - SER - ASN - ARG - LYS - LEU - MET - GLU - ILE - ILENH2
|
41
|
DOPAMINE
|
1
|
GROWTH HORMONE-RELEASING HORMONE (GHRH)
TYR - ALA - ASP - ALA - ILE - PHE - THR - ASN - SER - TYR - ARG - LYS - VAL - LEU - GLY - GLU - LEU - SER - ALA - ARG - LYS - LEU - LEU - GLN - ASP - ILE - MET - SER - ARG - GLU - GLN - GLY - GLU - SER - ASN - GLN - GLU - ARG - GLY - ALA - ARG - ALA - ARG - LEUNH2
|
44
|
GONADOTROPIN-RELEASING HORMONE (GnRH)
pyroGLU - HIS - TRP - SER - TYR - GLY - LEU - ARG - PRO - GLYNH2 -
|
10
|
SOMATOSTATIN
ALA - GLY - CYS - LYS - ASN - PHE - PHE - TRP - LYS - THR - PHE - THR - SER - SER – CYS S _____________________S
|
14
|
THYROTROPIN-RELEASING HORMONE
pyroGLU - HIS - PRONH2
|
3
|
Many axon terminals, however, do not abut directly on portal capillaries or terminate at some distance from the portal capillary plexus, may be to serve a modulatory role on other axon terminals rather than secrete into the portal plexus and explain the large numbers of peptides in the median eminence that either have no certain, direct action on anterior pituitary cells or cannot be measured in the portal blood (86). Axon terminals containing dopamine, for example, are located in close proximity to axon terminals containing GnRH (87) at the lateral margins of the external zone of the median eminence and can modulate the secretion of GnRH by presynaptic inhibition (88,89). Galanin containing axon terminals have also been observed to overlap with GnRH terminals in the lateral portion of the median eminence (90) but stimulate GnRH release from median eminence fragments (91). Although axo-axonal synapses are uncommon in the median eminence of most animal species studied using morphologic criteria (54), receptors for several different peptide hormones have been identified on axon terminals in the external zone suggesting that axo-axonal interactions can take place. Given the slow circulation time of blood perusing the median eminence (92), synaptic specialization in the median eminence may be unnecessary.
Alternatively, axons terminating at a distance from the portal capillaries may be held in reserve and only secrete to the anterior pituitary under certain physiological conditions. This phenomenon has been described for several peptides such as neuropeptide Y, whose concentration increases in portal capillary blood during an ovulatory surge to potentiate the action of GnRH on gonadotropin secretion (93,94). Similarly, VIP/PHI, which shows a minimal immunocytochemical staining pattern in the median eminence in the basal state, increases during suckling to stimulate prolactin release (95) and vasopressin markedly accumulates in the external zone following adrenalectomy (96). The anatomical correlate of these physiologic observations may be suggested by the work by King and Letourneau (97) on gonadotropin regulation in which GnRH-containing axon terminals in the median eminence can be found at different distances from the portal capillaries in intact animals’ vs gonadectomized animals. This indicates the potential for a dynamic association between axon terminals of the tuberoinfundibular system and the portal capillaries under specific physiologic conditions. Marked reorganization in the median eminence of several different peptide-containing axon terminals in the median eminence has also been observed following hypophysectomy (98).
A further complexity to the physiology of axon secretion in the external zone of the median eminence is the common occurrence of more than one peptide or transmitter coexisting in the same axon terminal. For example, TRH and preproTRH 160-169 coexist in the same axon terminals in the median eminence (99) and together have important potentiating effects on anterior pituitary TSH secretion (100). Galanin coexists with GHRH in the majority of GHRH-tuberoinfundibular neurons (101) and although does not stimulate growth hormone secretion by itself in dispersed anterior pituitary cells (102), when administered together with GHRH, it increases GH secretion over what can be achieved by GHRH alone (103). Rather than arise as a biosynthetic product of the same precursor molecule as preproTRH 160-169 and TRH, galanin and GHRH are derived from two separate gene products, expanding the possible sources for peptides that potentiate anterior pituitary secretion (104). The coexistence of substances in axon terminals may also help to coordinate the secretion of separate anterior pituitary hormones as has been proposed for VIP/PHI, neurotensin, and enkephalin in CRH-producing neurons (105) to coordinate the secretion of ACTH, GH and prolactin during stress (106).
In addition to axon terminals in the external zone of the median eminence, densely packed fibers that contain VIP and the nitric oxide-synthesizing enzyme, nitric oxide synthase (107) have been described on the ventral surface of the median eminence separated from the external layer (108). These fibers surround portal vessels and innervate smooth muscle of precapillary arterioles that supply the portal capillary plexus of the median eminence. Since both VIP and NO are potent vasodilators (109,110), these substances may play an important role in regulating the rate of blood flow to the median eminence and hence to the anterior pituitary, thereby exerting a separate level of control over anterior pituitary secretion. As opposed to axon terminals in the external zone of the median eminence that derive from the hypothalamus (see below), axons involved in regulation of portal blood flow appear to arise from other regions such as the sphenopalatine ganglion (107,108).
Consistent with the concept that the median eminence lies outside of the blood-brain barrier, claudin-5 and ZO-1, markers for tight junctions, are absent from vessels in the external layer (63).
Organum Vasculosum of the Lamina Terminalis (OVLT)
The OVLT is located in the midline of the lamina terminalis as part of the anterior wall of the third ventricle (Fig. 19). Its dorsal surface protrudes into the third ventricle cavity and its ventral surface is in direct contact with the prechiasmatic cistern. Thus, OVLT cells are in a position to be bathed by soluble factors in the CSF in both ventricular and cisternal spaces. In rodents, ultrastructural studies by Weindl et al (111) and Mitro and Palkovits (112) have described a variety of cell types in the OVLT, including specialized neurons, tanycytes, ciliated ependyma, and glial cells (113). Some of these cells send long processes to the periventricular space, whereas others establish specialized junctions and synaptic contacts or project outside the OVLT (113-115).
As in the median eminence, the OVLT contains fenestrated capillaries. They are derived from small branches of the preoptic artery that break up into a dense network of small vessels in the pia matter lining the external surface of the lamina terminals, and loop up towards the ventricular lumen (116). These vessels circumscribe interstitial spaces filled with cellular processes and secretory nerve endings that contain a number of neurotransmitter substances including atrial naturetic peptide, vasopressin, somatostatin, and GnRH (117), suggesting that like the median eminence, the OVLT subserves a neuroendocrine function. In contrast to the median eminence, however, blood from the OVLT does not drain into a portal plexus, but rather primarily to the medial preoptic region (118), suggesting a close functional interrelationship between the OVLT and this region of the hypothalamus. In addition, neurons in the OVLT project to the preoptic nucleus, subfornical organ, arcuate nucleus, supraoptic nucleus, medial thalamus and parts of the limbic system, primarily the cingulate, temporal and insular cortices. This anatomical organization, therefore, strategically places the OVLT in an ideal location to receive blood-born information and then transmit this information to specific regions of the brain. Accordingly, the OVLT has been implicated in mediating the febrile effects of circulating cytokines (see Thermoregulation). In addition, the OVLT is involved in osmoregulation and fluid balance through osmoreceptor cells that express the transient receptor potential vanilloid (TRPV) 1 gene (119), and respond to circulating levels of angiotensin II and relaxin (120,121). Its osmoregulatory role has been recently demonstrated in human volunteers subjected to excessive sweating using functional neuroimaging and blood flow distribution. These studies showed that the OVLT is co-activated with limbic regions well known to be involved in thirst consciousness after thalamic relay (cingulate and temporal cortex), whereas after water ingestion, prominent activation of the cortical satiety centers (insula) occurred (122). The OVLT is also densely innervated by axon terminals containing GnRH, originating from perikarya in the septum and areas surrounding the OVLT that presumably contribute to the regulation of pituitary gonadotropin secretion (123), perhaps through connections between the OVLT and the median eminence (124). In female rodents, these axons cross the OVLT en route to the median eminence to trigger pulsatile proestral release of pituitary gonadotropins (125), whereas in males, their gonadotropin-releasing hormone content is regulated by levels of circulating thyroid hormone (126,127). Direct connections between the OVLT and the median eminence have also been described (124).
Subfornical Organ (SFO)
The name of this circumventricular organ derives from its midline, anatomical location under the fornix (Fig. 18), at the point where the lamina terminalis joins the tela choroidea of the third ventricle (128). Embryologically, the SFO arises from the same part of the neural tube as the OVLT, and accordingly, have a similar microarchitecture and share common functions (129).
The SFO can be divided into two regions: a peripheral shell or “perimeter” that is rich in nerve endings arising from neurons intrinsic to the SFO but poor in blood capillaries, and a more densely packed center or “core” crowded with neuronal and glial perikarya and containing a dense vascular network of fenestrated and unfenestrated capillaries. In caudal portions of the SFO, capillaries are continuous with those of the choroid plexus (130). It is presumed that the “core” of the SFO is the locus for major hormonal receptor fields and fiber terminals of its afferent neuronal innervation, particularly the median preoptic nucleus, whereas the “perimeter” is the site of exit for SFO axons projecting to specific target regions in the hypothalamus including the preoptic nucleus, OVLT, supraoptic nucleus, paraventricular nucleus and lateral hypothalamus (131).
The SFO has an important role in coordination of fluid balance with blood pressure and drinking behavior, especially during hemorrhage and hypovolemia (132). The rich vasculature of the SFO allows circulating angiotensin II to stimulate intrinsic neurons (133) via angiotensin type 1 receptors (134). Through direct projections to the paraventricular nucleus, supraoptic nucleus and accessory magnocellular cell groups of the hypothalamus (135), SFO neurons induce release of vasopressin from the posterior pituitary (136), activate paraventricular nucleus neurons that descend to sympathetic centers of the spinal cord that regulate vasoconstriction (137), and possibly favor the release of vasoactive peptides like VIP from the anterior pituitary and a number of other neural sites related to fluid and blood pressure balance (138). Evidence for intrinsic production of angiotensin II in the SFO (139) and the antagonistic effects of galanin released from axon terminals that synapse on SFO neurons on angiotensin II-induced drinking behavior and vasopressin release (140), may also contribute to the regulation of fluid homeostasis. The presence of other peptides in the SFO and/or their receptors including obestatin, somatostatin and thyrotropin-releasing hormone, has suggested that the SFO might play a role in coordinating the ingestive behavior of liquids with solid food and the sleep cycle (141-143). This idea is also supported by the presence of leptin receptors in the SFO, and that their deletion abolishes the leptin-mediated increase in sympathetic outflow to the kidney (144). In addition, due to its connections with the preoptic nucleus and OVLT, the SFO is also involved in the regulation of thirst and locomotor behavior for drinking (145).
FIBER SYSTEMS
The fiber systems that link the hypothalamus to the rest of the brain are numerous and intricate, reflecting the importance of the hypothalamus as an integrating center for the rest of the brain. Due to the complexity of the fiber systems, however, it is impractical to individually describe each fiber pathway linking each nuclear group, particularly for the human hypothalamus in which nuclear boundaries and relative projections are less clear than in other mammals. Readers are referred to extensive reviews on this topic (146,147). We will describe only the major hypothalamic fiber systems with respect to their afferent and efferent connections to the periventricular, medial and lateral hypothalamic nuclear subdivisions.
Afferent Connections
Inputs to the mammalian hypothalamus arise primarily from the limbic system, brainstem reticular formation, thalamus, subthalamus, basal ganglia, retina and possibly the neocortex (Fig. 22). Afferents from the limbic system include 3 main fiber groups, the medial forebrain bundle, the stria terminalis, and the fornix. The medial forebrain bundle is located in the most lateral part of the hypothalamus and contains fibers originating from more than 50 nuclear groups in different regions of the brain including descending fibers from the olfactory and septal areas, and ascending fibers from the amygdaloid complex and substantia innominata, the latter forming the ventral amygdalofugal component of the ansa peduncularis. The stria terminalis originates in the amygdaloid complex, and the fornix in the hippocampus, both entering the rostral-medial hypothalamus close to the ventricular surface, and then arching into the substance of the hypothalamus to terminate along the entire extent of the hypothalamus.
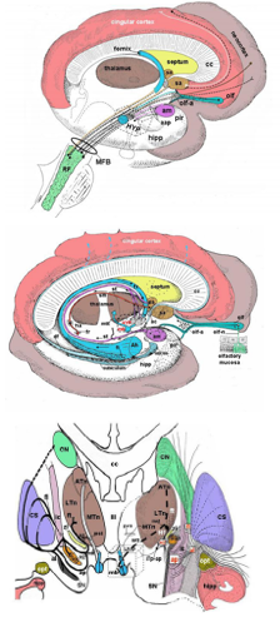
Figure 22. Overview of the major afferent pathways to the hypothalamus. (A) Schematic organization of medial forebrain bundle (MFB). Fibers afferent to the hypothalamus enter the lateral wall of the hypothalamus and are shown in different colors in relation to their anatomical source (amygdala, septal areas, olfactory areas, frontal neocortex). Reciprocal efferent connections from the hypothalamus to the same regions are shown by dotted black lines parallel to the colored lines. Pink fibers (and related reciprocal black dotted lines) indicate the amygdalofugal (and related amygdalopetal) components of the ansa peduncularis, entering the hypothalamus as a part of the medial forebrain bundle. The mammillary body and anterior column of the fornix are colored light blue and lie medial to the course of medial forebrain bundle. (B) Schematic organization of limbic afferents to the hypothalamus via the fornix (fx), stria terminalis (st), stria medullaris (sm), and olfactory tract. Axons enter the rostral portion of the hypothalamus before coursing throughout its entire extent. (C) Course of afferent fibers from the thalamus, subthalamus and zona incerta to the hypothalamus. Efferents from the hypothalamus coursing in the mammillo-subthalamic tract are also shown. On the right side of the image, a three-dimensional reconstruction shows the anatomical structures schematically depicted on the left side. aap = amygdalofugal and amygdalopetal components of the ansa peduncolaris; ac = anterior commissure; Ah = Ammon horn; al = ansa lenticularis; am = amygdala; ap = ansa peduncularis; ATn = anterior thalamic nucleus; cc = corpus callosum. CN = caudate nucleus; CS = corpus striatum; df = dentate fascia; fl = fasciculus lenticularis; fr = fasciculus retroflexus; ft = fasciculus thalamicus; fx = fornix; H1 = field H1 of Forel; H2 = field H2 of Forel; ha = habenula; hipp = hippocampus; HYP = hypothalamus; ic = internal capsule; iTp-ap = inferior thalamic peduncle of the ansa peduncularis; LTn = lateral thalamic nucleus; mb = mammillary body; MFB = medial forebrain bundle; mst = mammillo-subthalamic tract; MTn = medial thalamic nucleus; mtt = mammillo-thalamic tract; olf-a = olfactory area; olf-n = olfactory nerve; olf =olfactory tubercle; opt = optic tract; pir = piriform cortex; pvs = periventricular system; RF = reticular formation of the brainstem; sa = septal areas; SN = substantia nigra; Sub = subthalamus; zi = zona incerta; III = third ventricle. (From Toni R, Malaguti A, Benfenati F, Martini L: The human hypothalamus: a morphofunctional perspective. J Endocrinol Invest 27 (supp to n.6), 73-94, 2004.)
Afferents from the brainstem reticular formation include the dorsal longitudinal fasciculus (fasciculus of Schutz), the periventricular fiber system and the medial forebrain bundle. The dorsolongitudinal fasciculus receives primarily autonomic inputs from centers in the mesencephalic tegmentum (limbic midbrain area), reticular raphe nuclei in the pons and viscero-sensitive nuclei (e.g., the nucleus tractus solitarius) in the medulla oblongata. The periventricular system carries fibers ascending from both the central grey (including the raphe nuclei) and medial nuclei of the reticular formation in the mesencephalon or dorsal nucleus of the mesencephalic tegmentum (limbic midbrain area). Collectively, these fibers enter the hypothalamus close to the ventricular wall. The medial forebrain bundle also receives a well-defined fiber tract, the mammillary peduncle, originating from the medial nuclei of the mesencephalic reticular formation (limbic midbrain area). It courses ventrally in the cerebral peduncle (within the ventral tegmental area of Tsai), and then laterally to the mammillary bodies.
Afferents from the thalamus originate in nuclei of the median and medial thalamus, and course in the periventricular system and inferior thalamic peduncle of the ansa pednucularis (an extension of the medial forebrain bundle).
Afferents from the subthalamus are believed to originate in the nucleus subthalamicus and zona incerta, and directly enter the hypothalamus along the lateral aspect of the hypothalamic wall (148).
Afferents from basal ganglia (corpus striatum) arise from the nucleus accumbens, located in the ventral portion of the caudate nucleus, and via the substantia innominata, directly and indirectly reach the lateral portions of the hypothalamus.
Afferents from the retina reach the hypothalamus via the retino-hypothalamic tract, and travel through the optic chiasm to terminate in the suprachiasmatic nucleus. Finally, direct projections arise from the frontal cortex and course in the medial forebrain bundle to the most lateral part of the ventricular wall.
Efferent Connections
Outputs from the mammalian hypothalamus include fiber pathways to the anterior and posterior pituitary gland, limbic system, brainstem reticular formation, thalamus, subthalamus, basal ganglia, superior colliculi, substantia nigra, cerebellum, and neocortex (Fig. 23). With exception of projections to the pituitary gland, discussed in detail below (see Hypothalamic Tuberoinfundibular System and Hypothalamic Neurohypophysial Tract) and those directed to locomotor centers such as the optic tectum, susbstantia nigra, and cerebellum, in general, efferent fibers from the hypothalamus reciprocate its afferent fibers in a sort of feedback loop.
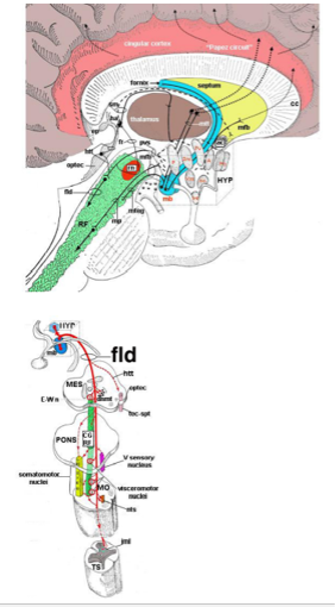
Figure 23. Overview of the major efferent pathways from the hypothalamus. (A) Connections with the limbic cortex, brainstem, thalamus and septum. (B) Course of hypothalamic fibers in the dorsal longitudinal fasciculus (fasciculus longitudinalis dorsalis) or fasciculus of Schutz, reaching autonomic and somatic centers in the brainstem and spinal cord. A = anterior hypothalamic nucleus; ac = anterior commissure; cc = corpus callosum; CGRF = central grey - reticular formation; Dm = dorsomedial nucleus; dnmt = dorsal nucleus of the mesencephalic tegmentum; E-Wn = nucleus of Edinger-Wepstal; fb = medial forebrain bundle; ep = epithalamus; fld = fasciculus longitudinalis dorsalis (fasciculus of Schutz); fr = fasciculus retroflexus; ha = habenula; HYP = hypothalamus; htt = habenulo-tectal tract; ilm = intermediate-lateral column of the spinal cord; inf = infundibular or arcuate nucleus; mb = mammillary body; MES = mesencephalon; mfb = medial forebrain bundle; MO = medulla oblongata; mteg = mammillo-tegmental tract; mp = mammillary peduncle; mtt = mammillo-thalamic tract; nts = nucleus tractus solitarius; optec = optic tectum; Po = preoptic area; PP = posterior nuclues; Pv = paraventricular nucleus; pvs = periventricular systems; sm = stria medullaris; so = supraoptic nucleus; RF = reticular formation; rn = red nucleus; tec-spt = tectospinal tract; TS = thoracic spine; Vm = ventromedial nucleus. (From Toni R, Malaguti A, Benfenati F, Martini L: The human hypothalamus: a morphofunctional perspective. J Endocrinol Invest 27 (supp to n.6), 73-94, 2004.)
Efferents to the limbic system include 2 major fiber groups. The first course in the medial forebrain bundle (lateral hypothalamus) and carries projections that ascend to the septal areas and descend to the amygdalo-piriform cortex complex. These latter fibers form the ventral amygdalopetal component of the ansa pedunclaris. The second, the stria terminalis system with its bed nucleus, is positioned medially, very close to the inner surface of the third ventricle, and projects to the amygdala.
Efferents to the brainstem reticular formation include 2 main fiber groups. The first is a dorsal and medial group formed by the dorsal longitudinal fasciculus and periventricular system. These axons descend to the brainstem to innervate visceral motor, sensory and somatic nuclei (oculomotor, trigeminal, facial, glossopharyngeal, vagus and accessory spinal nerves), and to autonomic sympathetic and parasympathetic preganglionic neurons in the spinal cord. The second courses in the medial forebrain bundle and gives off fibers that project to the medial nuclei of the mesencephalic reticular formation (limbic midbrain area) through the mammillary-tegmental tract and the mammillary peduncle.
Efferents to the thalamus include 3 main systems. The first is the mammillo-thalamic tract that links the posterior hypothalamus to the cingulate gyrus of the limbic cortex (a component of the “Papez circuit”). The second is the periventricular fiber system that projects to the medial (dorsomedian nucleus) and median (midline, intralaminar and reticular nuclei) thalamus and epithalamic habenula through the stria medullaris. The third is part of the medial forebrain bundle that course in the inferior thalamic peduncle of the ansa peduncularis to reach the medial thalamic nuclei.
Efferents to the subthalamic and basal ganglia region course in the lateral aspect of the ventricular wall in the mammillo-thalamic tract (mammillo-subthalamic tract) terminating in the field H1 of Forel, and in the substantia innominata (148,149). Efferents to superior colliculi, substantia nigra and cerebellum travel in either the stria terminalis and periventricular fiber system, or in the medial forebrain bundle as a part of the mammillary peduncle. Finally, efferents to the neocortex are carried laterally within the medial forebrain bundle.
Hypothalamic Tuberoinfundibular System
The hypothalamic tuberoinfundibular system comprises all neurons in the brain that send axonal projections to the external zone of the median eminence. Although the arcuate nucleus and inferior portion of the periventricular nucleus were thought primarily responsible for this pathway on the basis of silver stains (150), the relative paucity of myelin in these neurons and high density of perikarya in the medial and periventricular zones of the hypothalamus made it impossible to elucidate the full extent of the tuberoinfundibular system using this technique. In addition, the inability of pharmacologic ablation of the arcuate nucleus to significantly reduce the concentration of TRH and GnRH in the median eminence (151), made it likely that the origin of neurons contributing to the tuberoinfundibular system is considerably broader than just the medial basal hypothalamus. Using retrogradely transported marker substances that are taken up in axon terminals in the external zone of the median eminence and transported back to the cell body of origin, a detailed analysis of the tuberoinfundibular system has been possible (152-155).
Retrogradely labeled cells of the tuberoinfundibular system concentrate in four major hypothalamic regions: the arcuate nucleus, periventricular nucleus, paraventricular nucleus, and medial preoptic-septal region (Fig. 24). Within the arcuate nucleus the labeled cells accumulate in two distinct clusters (Fig. 24 G-I), a dorsomedial group of small to medium size neurons in the distribution of the dopamine-containing A12 group of Dahlstrom & Fuxe (156), and a basolateral group of medium sized cells that contain dopamine, GHRH, galanin, galanin-like peptide and neurotensin (156-161). Occasional enkephalin-producing neurons in the arcuate nucleus are retrogradely labeled from the median eminence (162), but the majority of these cells, as well as ACTH-producing neurons (personal observations), do not contain the retrogradely transported marker substance. These observations emphasize that only a small subset of chemically coded neurons in the arcuate nucleus project to the median eminence.
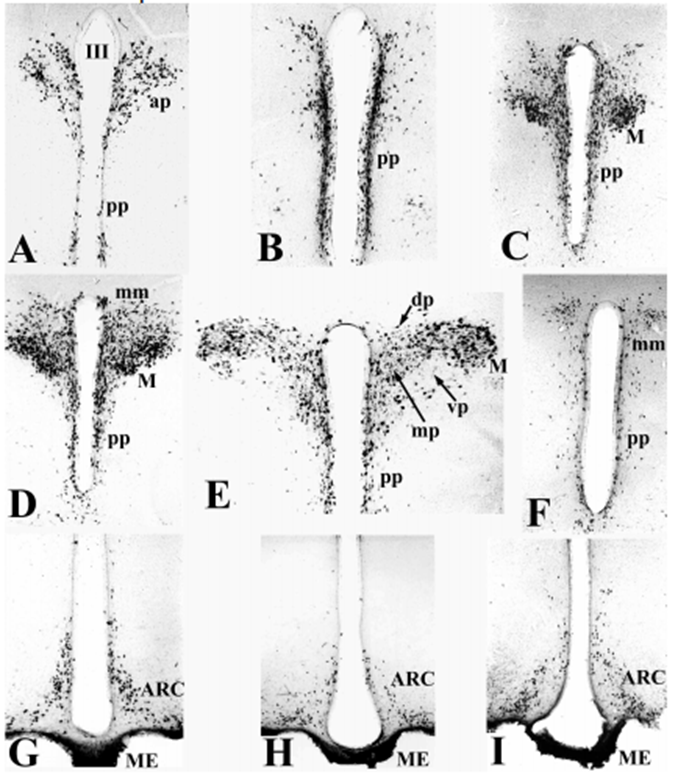
Figure 24. Coronal sections (rostral to caudal) of rat hypothalamus showing the regional distribution of neurons that accumulate a retrogradely transported marker substance injected into the external zone of the median eminence. Cells of origin of both the tuberoinfundibular pathway and hypothalamic neurohypophysial tract are identified due to diffusion of the tracer into the internal zone of the median eminence. (A-F) Level of the paraventricular nucleus (PVN); (G-I) level of the arcuate nucleus (ARC). III = third ventricle, AH = anterior hypothalamus, M = magnocellular division of the PVN, ME = median eminence, ap = anterior parvocellular subdivision, dp = dorsal parvocellular subdivision, mp = medial parvocellular subdivision, pp = periventricular parvocellular subdivision, vp = ventral parvocellular subdivision of the PVN.
In the periventricular nucleus (Fig. 24 A-F), a thin layer of retrogradely marked neurons in the subependymal neuropil contain somatostatin and dopamine (158,163). These retrogradely labeled cells can be identified even in the most rostral portions of the periventricular nucleus, but the majority concentrate between the middle of the optic chiasm and anterior portion of the median eminence (Fig. 24 B). Often cells interdigitate between the ependymal wall and even extend into the third ventricular space suggesting possible secretion into the CSF. Axonal projections from these cells to the median eminence is through a circuitous pathway that extends laterally into the lateral hypothalamus toward the ventral surface of the hypothalamus (retrochiasmatic area) and then medially to enter the median eminence, although some fibers also descend directly in the periventricular neuropil.
The most remarkable finding of studies using retrogradely transported marker substances from the median eminence is the massive accumulation of the marker substance in neurons of the paraventricular nucleus (Fig. 24 A-F). This winged-shaped nucleus at the dorsal margin of the third ventricle can be divided into two major portions based on the size of the neuronal perikarya, including a magnocellular division of large neurons and a parvocellular division of small to medium sized neurons (164). The parvocellular portion is located in the most medial portion of the nucleus adjacent to the ependymal wall of the third ventricle and can be broken down into several, smaller subdivisions shown in detail in Fig. 25. Retrogradely labeled cells of the tuberoinfundibular system are located primarily in the anterior, medial and periventricular subdivisions of the paraventricular nucleus with relatively few or no neurons in the dorsal, ventral and lateral parvocellular subdivisions. Many of these retrogradely labeled cells contain TRH, corticotropin-releasing hormone (CRH), enkephalin, somatostatin, and VIP (162,163,165-169). Not all neurons in the anterior, medial and periventricular parvocellular subdivisions project to the median eminence, however. This is particularly apparent for TRH neurons in the anterior parvocellular subdivision that cannot be retrogradely labeled by marker substances introduced into the median eminence (170). These neurons are also immunocytochemically distinct from hypophysiotropic TRH neurons in the medial and periventricular parvocellular subdivisions in that they do not co-express the peptide, cocaine and amphetamine-regulated transcript (CART) (171). The true, physiologic function of TRH neurons in the anterior parvocellular subdivision is not known.
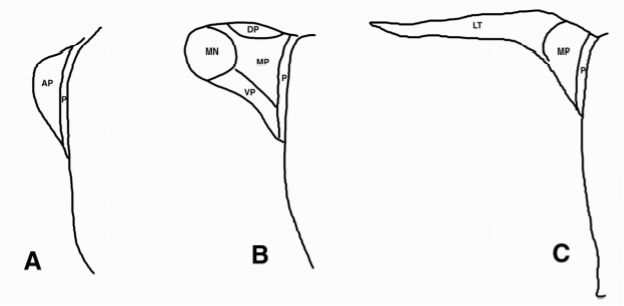
Figure 25. Schematic of the hypothalamic PVN showing major subdivisions. (A) Anterior, (B) Mid, and (C) Caudal levels. AP = anterior parvocellular subdivision, DP = dorsal parvocellular subdivision, LT = lateral parvocellular subdivision, MN = magnocellular division, MP = medial parvocellular subdivision, P = periventricular parvocellular subdivision, VP = ventral parvocellular subdivision.
Tuberoinfundibular neurons in the paraventricular nucleus project to the median eminence either by arching laterally and inferiorly through the lateral hypothalamus through the retrochiasmatic area before turning medially to terminate or by descending along the wall of the third ventricle to directly enter the median eminence. As the ependymal wall underlies both the paraventricular and periventricular nuclei and is likely permeable to the diffusion of CSF (68), these cells of the hypothalamic tuberoinfundibular system could be influenced by substances carried in the CSF or secrete directly into the CSF as an alternative way to reach the median eminence.
Finally, small, bipolar and multipolar, retrogradely labeled cells that can be immunostained with GnRH (169,172), are found in the rostral hypothalamus in the ventral wings of the diagonal band of Broca, lamina terminalis, medial septum, and medial preoptic nucleus, while few cells extend more caudally in the basolateral hypothalamus. In primates, however, retrogradely labeled GnRH cells are located more caudally in the basal hypothalamus (173). Axonal projections to the external zone of the median eminence occur either by joining the medial forebrain bundle in the lateral hypothalamus or along the wall of the third ventricle. The tendency for GnRH neurons of the tuberoinfundibular pathway to be more deeply embedded into the substance of the hypothalamus than is typical for the periventricular distribution of the majority of the hypothalamic tuberoinfundibular system relates to the embryologic origin of GnRH neurons from the nasal epithelium (174), as opposed to primordial cells in the walls of the third ventricle.
Although the bulk of tuberoinfundibular neurons arise from periventricular and medial portions of the hypothalamus, some brain stem neurons also have direct projections to the median eminence, explaining the presence of catecholamines in addition to dopamine in this structure. Retrogradely neurons can be identified in C1-C2 adrenergic neurons and A2 noradrenergic neurons (175), but since the tracer was injected into the bloodstream in this study, uptake could have occurred from other circumventricular organs in addition to the median eminence. Lesions of the brainstem, however, do result in degeneration of axon terminals in the median eminence (176).
Hypothalamic Neurohypophysial Tract
The hypothalamic neurohypophysial tract defines the neuronal system terminating in the posterior pituitary and is best known for its secretion of vasopressin and oxytocin into the peripheral circulation to regulate water balance (antidiuresis), milk ejection and uterine contraction (177). Neurons of this tract arise primarily from the magnocellular division of the paraventricular nucleus and the supraoptic nucleus (178), the latter situated as a cluster of cells dorsal and lateral to the optic chiasm (Fig. 26). The axon trajectory from magnocellular neurons to the posterior pituitary is by way of arching fibers extending laterally and inferiorly from the paraventricular nucleus above and below the fornix toward the supraoptic nucleus, where it gathers fibers from the supraoptic nucleus and continues medially along the base of the hypothalamus into the internal zone of the median eminence. Vasopressin-containing axon terminals have also been demonstrated in the external zone of the median eminence, particularly following adrenalectomy (179), but largely arise from a separate population of parvocellular neurons in the paraventricular nucleus that contain CRH (180). Vasopressin is a weak corticotropic factor but potentiates the secretion of ACTH in the presence of CRH (181) and is responsible for the ACTH rise following hypoglycemia (182).
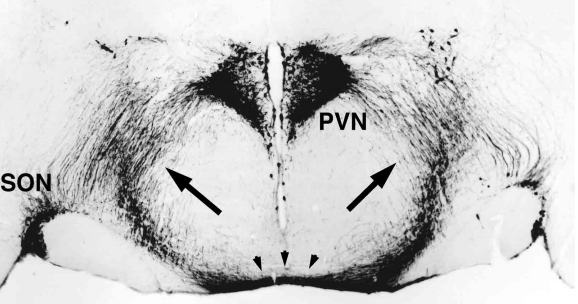
Figure 26. Organization of the hypothalamic neurohypophysial tract (arrows). Note arching fibers emanating from magnocellular neurons in the paraventricular nucleus (PVN) as they descend toward and join fibers emanating from the supraoptic nucleus (SON). The fiber tract converges in the midline at the base of the hypothalamus in the retrochiasmatic area (arrowheads) before entering the internal zone of the median eminence. III = third ventricle, F = fornix, OC = optic chiasm.
Magnocellular neurons of the paraventricular and supraoptic nucleus possess large perikarya and prominent dendrites that interdigitate with adjacent perikarya and dendrites, respectively, of other magnocellular neurons. These dendrites contain numerous hormone-laden neurosecretory granules that can be released by exocytosis (183) and may be important to coordinate the secretion of vasopressin or oxytocin from individual neurons in unison through somato-somatic and/or dendro-dendritic interactions, or alter the sensitivity of these neurons in response to a biologic stimulus such as suckling. Regulation of magnocellular neurons may also depend upon dynamic glial-neuronal interactions in response to specific stimuli, reducing or enlarging the cell to cell contact area between magnocellular neurons by retraction or extension of astrocytic processes that separate perikarya and dendrites (184), or to permit the formation of new synaptic contacts on magnocellular neurons (synaptic plasticity) (185).
In addition to vasopressin and oxytocin, magnocellular neurons of the hypothalamic neurohypophysial tract also produce and transport numerous other peptides to the posterior pituitary. Coexisting in vasopressin axon terminals are dynorphin, enkephalin, galanin, cholecystokinin, dopamine, TRH, VIP, neuropeptide Y, substance P, CRH, endothelin, pituitary adenylate pituitary cyclase-activating polypeptide (PACAP), secretin and glutamate, and in oxytocin terminals, dynorphin and proenkephalin A-derived µ-opioid peptides (186-190). A number of different neuropeptides are also carried into the posterior pituitary by axons from parvocellular neurons including GnRH, TRH, somatostatin, enkephalin, neurotensin CRH and dopamine (191). Furthermore, there is evidence that messenger RNA for vasopressin, oxytocin (192) and tyrosine hydroxylase (193) can be transported in axons of the hypothalamic neurohypophysial tract, particularly during osmotic stress.
The functional significance of numerous biologically active substances in axons from both magnocellular and parvocellular neurons, other than vasopressin and oxytocin, is of great interest. Since their concentration in the posterior pituitary is low, release into the peripheral circulation for action at a distant locus seems remote. Endothelin-1 may be an exception, however, where co-release with vasopressin into the periphery may assist the effect of vasopressin on water conservation by decreasing glomerular filtration rate (157). Other substances are likely involved in the regulation of vasopressin and oxytocin secretion by a paracrine or autocrine mechanism or by acting presynaptically on nerve endings in the posterior pituitary. Dopamine, for example, may be important in stimulating vasopressin release during an osmotic challenge (194). Neuropeptide Y has also been shown to enhance the secretion of vasopressin (195) and NPY Y2 receptors are present on nerve endings in the posterior pituitary in high density (196). Some neuropeptides in the posterior pituitary may act as trophic hormones (98), important to promote regeneration of its axon terminals following injury or to increase the proliferation of endothelial cells to promote changes in its vascularization (197).
There is considerable evidence, however, that some of the peptides in the posterior pituitary are destined for transport to the anterior pituitary via the short portal vessels, thereby utilizing the posterior pituitary as an accessory median eminence. The most convincing data that the posterior pituitary can influence anterior pituitary function comes from animal studies in which the posterior pituitary is surgically removed. Consequently, the blood flow to the anterior pituitary from the median eminence through the long portal veins is preserved, but the blood flow from the posterior pituitary through the short portal veins is disrupted. These animals have a number of abnormal neuroendocrine responses including a diminished ACTH response to stress (198), elevation in basal prolactin levels (193), and the loss of either suckling or estrogen induced prolactin release (199,200). These responses indicate the requirement of magnocellular derived-vasopressin or some other posterior pituitary secretagogue (CRH, oxytocin, dopamine, TRH, other prolactin releasing factor) to achieve normal physiologic responses.
Radiologic Anatomy
Magnetic resonance imaging (MRI) gives remarkable detail of the hypothalamus (Fig. 27) and thereby, has become the major radiologic tool to assess pathology in this region of the brain (201). While individual hypothalamic nuclear groups cannot be identified with this technique, some of the major fiber tracts that traverse the hypothalamus can be seen as high intensity signals, particularly in T-2 weighted images (202). These tracts include the fornix and the mammillothalamic tract, shown in Fig. 27B. Thus, using these fiber pathways as anatomical landmarks, it is possible to radiologically divide the hypothalamus into the two major subdivisions, the medial and lateral hypothalamic areas. In addition, in the most rostral portions of the hypothalamus, the anterior commissure is readily seen by MRI (Fig. 27A), and increased signal in the lateral hypothalamus is most likely due to the presence of the medial forebrain bundle (Fig. 27B-D).
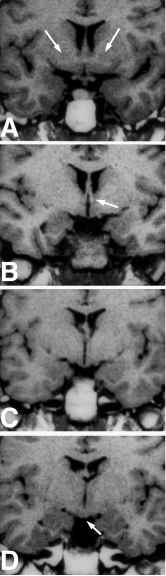
Figure 27. MRI of coronal sections through the hypothalamus. (A) Anterior hypothalamus corresponding to Fig. 16A showing location of the anterior commissure (arrows). (B) Mid hypothalamus corresponding to Fig. 16B showing location of the fornix (arrow). (C) Mid hypothalamus corresponding to Fig. 16C showing the optic tract. The fornix can sometimes also be visualized at this level. (D) Caudal hypothalamus corresponding to Fig. 16D at the level of the medial mammillary bodies (arrow). Sometimes the mammillothalamic tract can be visualized at this level.
Blood Supply
All arteries carrying blood to the hypothalamus are terminal branches of the circle of Willis, including the internal carotid, anterior cerebral, anterior communicating, posterior communicating, posterior cerebral and basilar arteries (Fig. 13). Curiously this arterial circle, named for Thomas Willis’s work published in Cerebri Anatome in 1664 (Fig. 28), had already been described between the end of the 16th century and beginning of the 17th century by the Italian anatomists, Fallopius and Casserio (203). These anatomists noted the existence of small arterial branches entering the floor of the third ventricle and surrounding the tuber cinereum in a position that is now well described as the anastomotic circuminfundibular plexus and prechiasmal anastomotic arteriolar-capillary plexus. These two anastomotic plexuses are highly developed in species such as Carnivora, Cetacea, Edentata and Ungulata (204) and likely represent the capillary system that Galen described as the rete mirabilis, given that he worked on animal species and not human brains and the anatomical differences between what he described and what is now recognized as the primary portal plexus (see below).
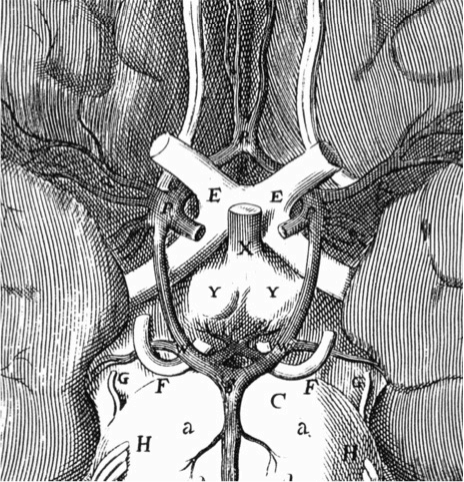
Figure 28. Original depiction of the arterial circle (or “polygon”) surrounding the hypothalamic infundibulum at the base of the brain, as shown in the Cerebri Anatome (1664) by Thomas Willis and drawn by the British architect, Cristopher Wren. Note the presence of branches to the mammillary bodies but absence of vessels to the infundibulum. (Courtesy of the Library of the Department of Human Anatomy of the University of Parma, Italy.)
The vascular supply to the hypothalamus has been extensively studied in man using three-dimensional casts of the hypothalamic vessels (205). These studies demonstrate that the arterial supply is compartmentalized with respect to three rostro-caudal regions of the hypothalamus, and thus, can be separated into anterior, intermediate and posterior arterial groups (Table 8). However, only the anterior hypothalamic region is vascularized by a single arterial group (the anterior arterial group), whereas the remainder of the hypothalamus receives blood from both the intermediate and posterior arterial groups. The preoptic and anterior hypothalamus are primarily supplied by the anterior cerebral and anterior communicating arteries, the tuberal region by the posterior communicating artery, and the mammillary region by the posterior communicating, posterior cerebral, and basilar arteries. This organization is consistent with the clinical observations that occlusion of the anterior choroidal artery, which has anastomotic branches with the posterior communicating artery, results in damage to the tuberal and mammillary regions of the hypothalamus, whereas occlusion of the thalamoperforate artery, a branch of the posterior cerebral artery, results in damage to the mammillothalamic tract and related thalamic nuclei (205).
Table 8. Arterial Groups that Supply Hypothalamic Nuclei
|
ANTERIOR GROUP
internal carotid, anterior cerebral and posterior communicating arteries
|
INTERMEDIATE GROUP
posterior communicating artery
|
POSTERIOR GROUP
posterior communicating, posterior cerebral and basilar arteries
|
AHA
|
ARC
|
LHA
|
MPA
|
DMN
|
LMN
|
PV
|
LHA
|
MMN
|
PVN
|
LMN
|
PN
|
SCN
|
LTN
|
PV
|
SON
|
MMN
|
SMN
|
|
PN
|
|
|
PV
|
|
|
SMN
|
|
|
VMN
|
|
AHA = anterior hypothalamic area, ARC = arcuate nucleus, DMN = dorsomedial nucleus, LHA = lateral hypothalamic area, LMN = lateral mammillary nucleus, LTN = lateral tuberal nucleus, MMN = medial mammillary nucleus, MPA = medial preoptic area, PN = posterior hypothalamic nucleus, PV = periventricular nucleus, PVN = paraventricular nucleus, SCN = suprachiasmatic nucleus, SMN = superamammillary nucleus, SON = supraoptic nucleus, VMN = ventromedial nucleus
As previously described, the blood supply to the median eminence is complex. In all mammals, including humans, fine branches of the superior hypophysial artery give rise to a capillary plexus, the primary portal plexus of the infundibulum, which is composed of capillary loops in the external zone of the median eminence (external plexus) and that penetrate as pillars vertically toward the ventricular floor to establish the internal plexus. At this level, a subependymal capillary network can also be recognized in association with the basal membrane of the ependymal cells. Blood flows from the external to the internal and then back to the external plexus to end in the long portal vessels that reach the anterior pituitary, or from the subependymal network into hypothalamic capillaries of the anterior or intermediate arterial groups (206). In some species, bidirectional transport of substances has been described in the portal capillary system, allowing the transport of anterior pituitary substances to the external plexus (207), thus supporting the original hypothesis of Popa and Fielding that in the human brain, blood flow in the portal capillary system can be from the pituitary to the hypothalamus (208). The external plexus is tangential to the ventral surface of the infundibulum and is composed of vessels organized in geometrical arrays (hexagonal in rodents, much more complex in humans), whose central spaces are filled with neuroendocrine axons constituting functional units, called microvascular domains (206) or medianosomes (209). Neurohemal contacts are established by these axons and by tanycyte processes at the level of both plexuses, whereas neurohypophysial fibers course between the two vascular plexuses without contacting them, en route to the posterior lobe of the pituitary. This “double-plexus” system provides amplification of the surface area in contact with tuberoinfundibular axons in a given microvascular domain.
Venous drainage from the rest of the hypothalamus is collected into the anterior cerebral, basal, and the internal cerebral veins, ultimately reaching the great vein of Galen. In general, the anterior cerebral and basal veins drain the majority of the hypothalamus, whereas the internal cerebral vein collects blood from the dorsal portions of the hypothalamus (Table 9).
Table 9. Venous Drainage from Hypothalamic Nuclei
|
Anterior Cerebral and Basal Veins
|
Internal Cerebral Vein
|
AHA
|
DMN
|
ARC
|
LHA
|
LHA
|
LMN
|
LMN
|
MMN
|
LTN
|
PV
|
MMN
|
PVN
|
MPA
|
SMN
|
PN
|
|
PVN
|
|
SCN
|
|
SMN
|
|
SON
|
|
VMN
|
|
AHA = anterior hypothalamic area, ARC = arcuate nucleus, DMN = dorsomedial nucleus, LHA = lateral hypothalamic area, LMN = lateral mammillary nucleus, LTN = lateral tuberal nucleus, MMN = medial mammillary nucleus, MPA = medial preoptic area, PN = posterior hypothalamic nucleus, PV = periventricular nucleus, PVN = paraventricular nucleus, SCN = suprachiasmatic nucleus, SMN = superamammillary nucleus, SON = supraoptic nucleus, VMN = ventromedial nucleus
Glymphatic System and Dural Sinus-associated Lymphatics
Recent studies in rodents have revealed that fluorescent tracers injected into the arachnoid cisternae can travel along a perivascular network of channels coursing throughout the brain, the hypothalamus included, and contain cerebrospinal fluid (CFS), water, food metabolites (primarily lipids) and proteins arising from tissue degradation/inflammation (e.g., β-amyloid), coined the glymphatic system (210). Although these findings replicate similar observations made more than 30 years ago using, horseradish peroxidase in dogs and cats (211), it is only recently that it became clear that these channels do originate as a continuation of the subarachnoid cisternae around the arteries dividing inside the brain parenchyma otherwise known as Virchow-Robin spaces. Virchow-Robin spaces are externally lined by the glia limitans that gives rise to a pavement underneath the basal lamina of the perivascular leptomeningeal cells facing the brain parenchyma, whereas a symmetrical leptomeningeal lining embraces the basal lamina of the smooth muscle, arterial cells. Once the arteries enter the depth of the brain matter to become penetrating arterioles and capillaries, they lose both their muscular sheath and perivascular leptomeninges, but a narrow interstitum persists between the basal lamina of the vascular endothelial cells and that of the original subarachnoid cells. The latter basal lamina is ensheated by the endfeet of aquaporin4 (AQP4)-expressing astrocytes that form the external boundaries of the glymphatic interstitium, where they regulate the passage of water and intercellular solutes from the interneuronal spaces into the perivascular CSF, and vice versa. The absence of endothelial tight junctions in circumventricular organs (63) such as the median emince, amplifies the opportunity for reciprocal exchange with blood-born substances. The liquid bulk is passed into a connected perivenular and perivenous space (212) that then drains into the extracranial perivenous lymphatics (213) (Fig. 29).
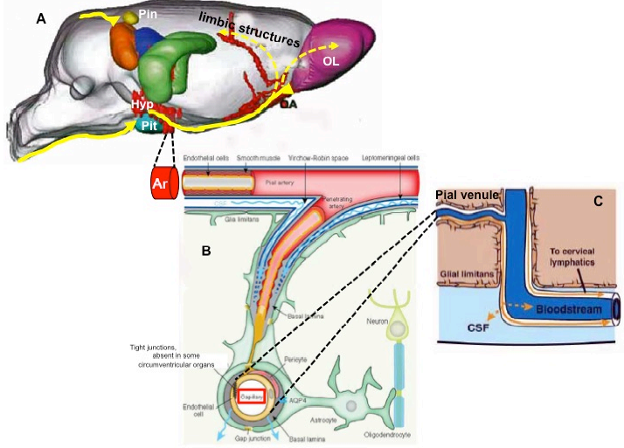
Figure 29. Composite of different drawings schematizing the 3D organization of the brain glymphatic system with particular reference to the hypothalamic-pituitary unit. A) Distribution by magnetic resonance imaging of paramagnetic contrast (yellow arrows) in paravascular channels of the rat hypothalamus (HYP), pituitary (Pit), and pineal (Pin) recesses following injection into the cisterna magna (adapted from ref. 30). A contingent of glymphatic flow connects the medial-basal hypothalamus to the limbic structures and olfactory area (OA) and lobe (OL), ensuring a wide interplay by local soluble materials (volume transmission); B) Structure of the glymphatic channels (adapted from ref. 212): pial arteries (Ar) coursing in the subarachnoid space are surrounded by CSF that flows in the perivascular space, termed the Virchow–Robin space. The arteriolar tunica media is isolated from the CSF by a lining of leptomeningeal cells; however, the CSF-containing Virchow–Robin spaces progressively narrow and finally disappear at the level of capillaries (red rectangle). The CSF continues its flow into perivascular spaces provided only by the extracellular matrix of the basal lamina. Astrocytic vascular endfeet expressing aquaporin-4 (AQP4) surround the entire vasculature and form the boundary of the perivascular spaces. These astrocytes may transfer information carried by solutes in the glymphatic system to surrounding glial cells and neurons, and vice versa; C) the glymphatic spaces are in continuity with venules and veins (adapted from ref. 210), allowing for glymphatic drainage either into sinus-associated dural lymphatics and venous brain sinuses or exiting the brain into perivenular lymphatics and lymph nodes.
Remarkably, the fluid dynamics in the hypothalamic glymphatic channels is affected by alterations in carbohydrate metabolism, such as diabetes mellitus, as a result of enlargement of the paravascular spaces by structural arteriolar and/or venular damage (214). This suggests a potential role for the hypothalamic glymphatic network in regulating the intrahypothalamic exposure of glucose, free fatty acids, and proteins to relevant hypothalamic nuclei involved in the regulation of appetite and satiety (See section on Appetite and Satiety). Similar, transthyretin-coupled thyroid hormones reaching the CSF from the choroid plexus (215) might enter the hypothalamic glymphatic system and act as an amplifier of a thyroid-dependent, volume transmission in the hypothalamus (216), influencing a large array of hypothalamic homeostatic neurons (see section on Functional Anatomy of Hypothalamic Homeostatic Systems).
The glymphatic channels are in position to merge with dural lymphatics. The latter were originally described in the dura mater and pia mater in human cadavers in 1787 by the Italian anatomist, Paolo Mascagni (217), and recently rediscovered in rodent models (218,219) as an unexpected anatomical novelty (220), challenging the concept of brain parenchyma as a site of “immune privilege”. Endothelial cells of these conduits express markers of the lymphatic vessels including LYVE1, PROX1 and VEGFR3, and course parallel to both the main dural sinuses (sagittal and transverse) and dural middle meningeal arteries, the latter laying adjacent to the dural boundaries of the cavernous sinus where dural lymphatics might establish a direct cavernous connection (30). Therefore, it has been suggested that the perivascular pathways of the glymphatic system and meningeal lymphatic vessels should be considered as serial elements of an interconnected circuitry for immune surveillance of the brain by the immune system, presumably allowing brain antigens access to lymph nodes outside the brain parenchyma (221). Such a circulatory pathway may contribute to the pathogenesis of inflammatory and autoimmune conditions of the hypothalamic-pituitary unit (222,223) including lymphocytic hypophysitis and diabetes insipidus (Fig. 29).
FUNCTIONAL ANATOMY OF HYPOTHALAMIC HOMEOSTATIS SYSTEMS
Regulation of Hypophysiotropic Neurons
The secretion of hypothalamic releasing and inhibitory hormones from axon terminals of tuberoinfundibular neurons into the portal capillary system is dependent upon several layers of control that can be exerted directly on the perikarya and/or processes of these neurons. For one, neurons of the tuberoinfundibular system can be modulated by substances circulating in the bloodstream that either pass the blood-brain barrier because they are fat soluble steroids or small molecules, or access tuberoinfundibular neurons via the cerebrospinal fluid due to the periventricular location of many tuberoinfundibular neurons and poor development of tight junctions between ependymal cells in these regions (63). Feedback effects of thyroid hormone, for example, occur directly on TRH-producing neurons within the paraventricular nucleus as demonstrated by the ability of a microcrystalline implant of T3 adjacent to the paraventricular nucleus to prevent the hypothyroid-induced increase in TRH biosynthesis on that side but not the opposite side (224,225). In addition, tuberoinfundibular neurons receive numerous axosomatic and/or axodendritic contacts from local interneurons and/or other regions in the brain that contain a variety of chemical messengers that contribute to intercommunication between specific neuronal groups or are important in establishing the set point at which the hypophysiotropic substances are secreted in response to hormonal feedback signals. To demonstrate how the CNS can exert regulatory control over hypophysiotropic neurons, examples of modulation of GH secretion and regulation of the hypothalamic-pituitary-adrenal (HPA), hypothalamic-pituitary-thyroid (HPT), and reproductive axes are given below.
MODULATION OF GHRH/SRIF TUBEROINFUNDIBULAR NEURONS
A well-studied example of local afferent influences on the activity of tuberoinfundibular neurons is demonstrated by the hypothalamic regulatory system involved in the control of GH secretion. The pattern of GH secretion is episodic, showing a regular periodicity of one pulse every 2 to 4 hours and low or undetectable trough values (226). This rhythm is the result of the control by two separate components of the tuberoinfundibular system, including GHRH-producing neurons (stimulatory) in the basolateral portion of the arcuate nucleus and somatostatin-producing neurons (inhibitory) in the periventricular nucleus, each secreting into the portal capillary plexus. To coordinate this rhythmic secretion, reciprocal axonal connections between these two populations of neurons may be necessary (Fig. 30). In this manner, somatostatin neurons receive direct, stimulatory inputs from GHRH neurons while GHRH neurons receive direct, inhibitory inputs from somatostatin-containing neurons, Treatment of hypothalamic cultures with somatostatin inhibits GHRH, whereas treatment with GHRH induces somatostatin release (227). In addition, both GH and IGF-1 cross the blood-brain barrier with GH increasing somatostatin secretion into the portal capillary system, whereas IGF-1 both increases somatostatin and inhibits GHRH, contributing to a finely tuned regulatory system (228,229). GH secretion can also be modulated by a number of other factors including glucose and free fatty acids circulating in the bloodstream, but also neurotransmitters and peptides intrinsic to the central nervous system, the latter acting primarily on somatostatin and/or GHRH producing tuberoinfundibular neurons. For example, GH receptors are expressed by the majority of NPY neurons in the hypothalamic arcuate nucleus that show c-Fos expression in response to GH administration and are known to innervate periventricular somatostatin neurons and increase somatostatin secretion (230). The rise of GH during sleep is probably mediated by cholinergic pathways suppressing somatostatin secretion (231). Stress and sepsis can also be associated with a rise in GH levels mediated by catecholamines by increasing GHRH (232). The precise origin of the neurons giving rise to these neuromodulators, however, is not known. Glutamate may also be of importance by increasing the secretion of GHRH as N-methyl-D-aspartate increases GH secretion (233), and this can be attenuated by GHRH antibodies (234). In addition, the majority of GHRH neurons in the arcuate nucleus are contacted by axons expressing the glutamate transporter 2 (VGLUT2) (235), a selective marker for glutamatergic elements. PACAP also increases GH secretion, but PACAP knock-outs do not have disturbance in GH release (236,237). Multiple other peptides have also been shown to have either a stimulatory or inhibitory effect on GH secretion but their physiological significance is unknown (238).
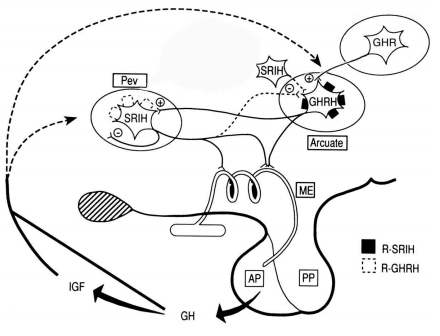
Figure 30. Schematic representation of the interactions between somatostatin (SRIH)-producing neurons in the hypothalamic periventricular nucleus (Pev) and growth hormone releasing hormone (GHRH)-producing neurons in the arcuate nucleus. Note that in addition to projections to the external zone of the median eminence, these neurons may also have reciprocal connections. Ghrelin (GHR) may also influence GH secretion by acting directly on somatotrophs or through stimulatory effects on GHRH neurons. AP = anterior pituitary, GH = growth hormone, IGF = insulin-like growth factor, ME = median eminence, PP = posterior pituitary. R-SRIH and R-GHRH correspond to receptors for the respective peptides. (Adapted from Epelbaum J: Intrahypothalamic neurohormonal interactions in the control of growth hormone secretion. Functional Anatomy of the Neuroendocrine Hypothalamus, Wiley, Chichester, 1992; pp 54-68.)
A relatively new and potentially exciting chapter in the understanding of the physiology of GH secretion has been the discovery of ghrelin, the most potent (on a molar basis) GH secretagogue known to man (239). Ghrelin circulates in the bloodstream, secreted primarily from the stomach, but is also produced by neurons in the hypothalamic arcuate nucleus (240). When administered as a single intravenous dose, it induces an acute release of GH, and when administered continuously, it increases 24h pulsatile secretion of GH (241). Although the anterior pituitary somatotrophs contain receptors for ghrelin, and ghrelin can directly induce GH secretion, it is more likely that ghrelin exerts its main effects in the hypothalamus by triggering GHRH secretion (242), as pulsatile patterns of plasma or CSF ghrelin do not correlate well to circulating GH concentrations (243). Ghrelin levels fall in response to rising levels of GH, providing evidence for a gastro-hypophysial feedback loop (244). Other external and metabolic signals also can modulate GH secretion such as the decrease in blood glucose following a carbohydrate-rich meal, hypoglycemia, and a high intake of protein that are believed to function through a common mechanism of somatostatin withdrawal and disinhibition of GH secretion (245).
MODULATION OF CRH TUBEROINFUNDIBULAR NEURONS
Analogous to the hypothalamic-pituitary-thyroid axis alluded to above and discussed in greater detail in the following section, the hypothalamic-pituitary-adrenal axis is similarly modulated by direct feedback effects on hypophysiotropic CRH neurons by a circulating hormone, in this case glucocorticoids. Removal of the adrenals results in marked upregulation of CRH mRNA selectively in the PVN. Glucocorticoids can also exert additional, rapid signaling on hypophysiotropic CRH neurons via endocannabinoid-mediated suppression of synaptic excitation, in which 2-arachidonooyl-glycerol [2-AG] and N-arachidonoylethanolamine [AEA] from CRH neurons inhibit the release of glutamate from neurons synapsing on hypophysiotropic CRH neurons (246).
Afferent input to tuberoinfundibular neurons from distant loci in the brain, howere, is another important regulatory mechanism over hypophysiotropic function and is one way that tuberoinfundibular neurons are integrated with other functions of the brain. The parvocellular subdivision of the paraventricular nucleus receives direct, dense, afferent input from autonomic centers in the lower brain stem including the nucleus of the tractus solitarius (NTS), dorsal motor nucleus of the vagus (DMNv) and several catecholamine groups in the dorsal and ventral lateral medulla, carrying visceral sensory information primarily from the abdomen and thorax through the vagus and glossopharyngeal nerves (247). At least part of this projection is noradrenergic but other substances such as neuropeptide Y (248), activin (249), and GLP-1 (250) are also carried in these fibers, some coexisting in catecholaminergic neurons. After traversing through the medial forebrain bundle in the lateral hypothalamus, axons containing catecholamines have been observed to make numerous synaptic contacts with CRH-producing neurons in the paraventricular nucleus (251,252) and to induce the secretion of CRH primarily via α1 adrenergic receptors (253). In this manner, sensory information from the periphery (e.g., heart rate, blood pressure as during hemorrhagic shock) has the potential to alter the set point for the secretion of hypophysiotropic CRH using norepinephrine as the central mediator, and thereby increase circulating levels of glucocorticoids.
In a similar fashion, increased secretion of glucocorticoids in response to infection or inflammation is due to the activation of catecholaminergic neurons of the NTS (A2 noradrenergic, C2 adrenergic) and rostral ventrolateral medulla but initiated by endotoxin and proinflammatory cytokines such as interleukin-1 (IL-1) (254). Under these circumstances, the set point for feedback inhibition of hypophysiotropic CRH secretion is altered to allow the powerful immunosuppressant action of glucocorticoids to limit the severity of the inflammatory response (255). If the ascending catecholamine pathway to the PVN is transected, the ability of IL-1 to increase CRH mRNA in PVN neurons is reduced (256). It is proposed that IL-1 exerts its effect on endothelial cells and/or perivascular microglia at the blood-brain interface, resulting in activation of cyclooxygenase-2 (the rate limiting enzyme for the formation of prostaglandins), the release of prostaglandin E2 (PGE2) into the surrounding tissue, and ultimately activation of catecholaminergic neurons through prostaglandin receptors (257). This hypothesis is supported by the demonstration that focal injection of PGE2 into the medulla reproduces the activating effects of IL-1 on CRH neurons in the PVN (258). Alternatively, cytokines may exert their effects on vascular cells at the blood-brain-barrier directly within the PVN, itself, or after penetrating the blood-brain barrier at circumventricular organs such as the OVLT, and then transmitting the information through neural pathways that interconnect these structures with the PVN (257, see Thermoregulation below).
Neurogenic stress also leads to resetting of the HPA axis and similarly characterized by elevated circulating glucocorticoid levels and increased CRH gene expression in hypophysiotropic neurons (254). However, the mechanism would appear to be vastly different than that described above as indicated by persistent HPA activation under these conditions despite disruption of the ascending catecholaminergic pathways to the PVN (259). CRH neurons receive inputs from other portions of the brain such as the forebrain limbic system, and surgical ablation of hippocampal efferents to the hypothalamus (260) or lesions in the bed nucleus of the stria terminalis (261) increase the concentration of CRH mRNA in the paraventricular nucleus. In addition, activation of NPY neurons originating in the hypothalamic arcuate nucleus may contribute to increased CRH cellular activity induced by insulin hypoglycemia (262,263). Interactions among axon terminals containing GABA, glutamate and endocannabinoids may also contribute to modulation of stress-induced activation of CRH neurons (264). Thus, while the end result to increase circulating levels of glucocorticoids is similar in all stress paradigms, depending upon the type of stress, different regions of the brain are recruited to allow resetting of the HPA axis. The mitogen activated protein (MAP) kinase signaling pathway may be a common final mechanism in hypophysiotropic CRH neurons that links a variety of different stimuli that activate the HPA axis to an increase in CRH gene expression (265-267). Stress and glucocorticoid-induced synaptic plasticity of the neural circuitry regulating hypophysiotropic CRH neurons may also contribute to regulation of the HPA axis, particularly in the face of chronic stress (264).
The circuitry described above that allows resetting of the HPA axis is illustrated in Fig. 31.
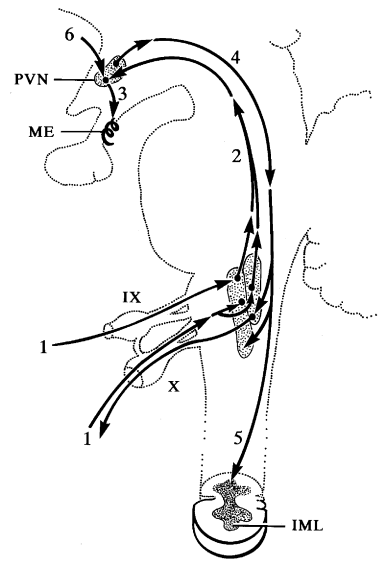
Figure 31. Schematic diagram showing autonomic regulation of corticotropin-releasing hormone (CRH) neurons of the hypothalamic tuberoinfundibular tract. (1) Visceral sensory information carried by the glossopharyngeal (IX) and vagus (X) nerves terminates in lower brain stem autonomic centers which (2) project to CRH neurons in the paraventricular nucleus (PVN), (3) modulating the secretion of CRH into the portal capillary system in the median eminence (ME). Descending pathways from the PVN reach autonomic centers in the (4) lower brainstem and (5) spinal cord and can influence the autonomic nervous system. (6) CRH neurons also receive direct input from the limbic system via bed nucleus of the stria terminalis. IML = intermediolateral cell column of the spinal cord. (Schematic diagram based on rat data and modified from Sawchenko PE, Swanson LW, Science 1981; 214:685-687.)
MODULATION OF TRH TUBEROINFUNDIBULAR NEURONS
The secretion of hypophysiotropic TRH is primarily regulated by negative feedback regulation by thyroid hormone mediated by the beta 2 isoform of the thyroid hormone receptor (TRβ2) expressed on these neurons (268). As T3, alone, is not sufficient to normalize TSH expression in the PVN except when administered in thyrotoxic doses (269), under normal conditions, feedback inhibition is dependent upon the conversion of T4 to T3. Hypophysiotropic TRH neurons, however, do not contain the enzyme necessary for T4 to T3 conversion, type 2 iodothyronine deiodinase (D2). Rather, it is believed that T3 production is mediated by tanycytes, specialized ependymal cells lining the third ventricle. These cells not only contain D2, but also express the thyroid hormone transporters, OATP1 and MCT8, on their surface. It is proposed that T3 released from tanycytes contribute to feedback regulation by thyroid hormone viamechanisms further elaborated below (270). As noted previously, tanycytes also express the TRH degrading ecto-enzyme, pyroglutamyl peptidase II (PPII), in keeping with the important role of tanycytes in control of the hypothalamic-pituitary-thyroid axis. Hypophysiotropic TRH neurons also express the vesicular glutamate transporter 2 (VGLUT2), whereas tanycytes the line the median eminence express AMPA and kainite receptors and glutamate transporters and are depolarized by glutamate and by optogenetic activation of TRH axons in the median eminence (271). As the majority of hypophysiotropic TRH neurons express the type 1 cannabinoid receptor (CB1) mRNA and contain punctate CB1-immunoreactive signal in their axon varicosities, tanycytes in close association with TRH-containing axon terminals in the median eminence contain the endocannabinoid synthesizing enzyme, diacylglycerol lipase α (DAGLα), antagonizing CB1 or inhibiting DAGLα stimulates TRH release in median eminence explants and inhibition of glutamate signaling markedly decreases the 2-AG content of the median eminence (271), the existence of a microcircuit in the median eminence is suggested in which the activity of TRH axons stimulate endocannabinoid synthesis in tanycytes, whereas the tanycytes restrain the delivery of TRH to the anterior pituitary via the endocannabinoid system. This interaction may have importance in the regulation of pulsatile secretion of TRH and provide and extra flexibility to the control of TRH release.
Elucidation of the mechanisms by which the hypothalamic-pituitary-thyroid (HPT) axis responds to fasting provides another excellent example of how afferent input from neurons arising outside of the PVN can influence the secretion of hypophysiotropic neurons. Similar to the feedback mechanisms controlling the adrenal axis, maintenance of normal thyroid function is dependent upon a negative feedback control system. Circulating levels of thyroid hormone (T4 and T3) influence the biosynthesis and secretion of TRH in hypophysiotropic neurons in the PVN (Fig. 32) and TSH in the anterior pituitary (225), although a role for thyroid hormone-induced upregulation of the TRH degrading enzyme, pyroglutamyl peptidase II, expressed in tanycytes has also been proposed by regulating the amount of TRH released into the portal system (272). In response to fasting or infection, however, this normal homeostatic system is altered in a way that is presumably beneficial for survival. Under these circumstances, there is a fall in circulating thyroid hormone levels but a seemingly paradoxical reduction in TRH gene expression in the PVN (Fig. 33), reduced secretion of TRH into the portal blood and low or inappropriately normal plasma TSH (273-276), rather than the anticipated increase in all of these parameters as seen in primary hypothyroidism mediated by a decline in circulating levels of leptin (see below for mechanism). Thus, during fasting, the normal feedback mechanism described above is overridden, and a state of central hypothyroidism is transiently induced. Presumably, by reducing thyroid thermogenesis and preserving nitrogen stores, this mechanism is an important adaptive response to reduce energy expenditure until the adverse stimulus is removed (277).
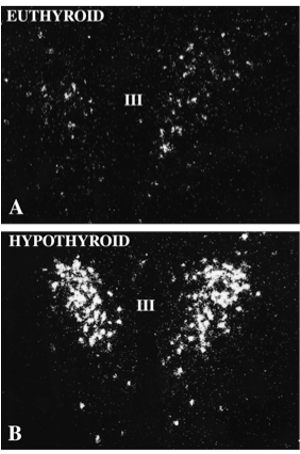
Figure 32. High magnification in situ hybridization autoradiographs of proTRH mRNA in the paraventricular nucleus (PVN) of a (A) euthyroid and (B) hypothyroid animal. Note marked increase in TRH mRNA when circulating levels of thyroid hormone fall.
The HPT axis is primarily modulated by afferent input derived from the hypothalamus, itself. At least two anatomically distinct populations of neurons in the arcuate nucleus with opposing functions, proopiomelanocortin (POMC)-producing neurons that also co-express cocaine and amphetamine-regulated transcript (CART), and NPY-producing neurons that co-express agouti related peptide (AGRP), appear to be responsible (174,278-280). Both neuronal populations express receptors for the white adipose tissue-derived circulating hormone, leptin, and project to hypophysiotropic TRH neurons in the PVN through a monosynaptic, arcuate-PVN pathway (281-283). Alpha-MSH, a translation product of POMC, and CART (originally described as a mRNA induced in the striatum following psychostimulant drug administration) both induce transcription of the TRH gene in hypophysiotropic neurons (163,278), whereas NPY and AGRP are inhibitory (279,280), NPY via direct effects on Y1 and Y5 receptors on TRH neurons (269), and AGRP by antagonizing α-MSH at melanocortin receptors (285). Thus, during fasting when circulating levels of leptin decline, expression of the genes encoding POMC and CART are reduced simultaneously with a marked increase in the genes encoding NPY and AGRP (286,287), effectively lowering the threshold of feedback inhibition of hypophysiotropic TRH by circulating levels of thyroid hormone.
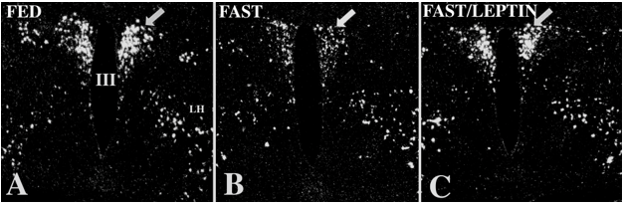
Figure 33. In situ hybridization autoradiographs of proTRH mRNA in the PVN (arrow) of (A) normal fed and (B) fasting animals. Note the marked reduction in hybridization signal by fasting. (C) ProTRH mRNA levels are restored to normal in fasting animals administered leptin. (From G. Legradi, C.H. Emerson, R.S. Ahima, J.S. Flier, R.M. Lechan, Leptin Prevents Fasting-Induced Suppression of Prothyrotropin-Releasing Hormone Messenger Ribonucleic Acid in Neurons of the Hypothalamic Paraventricular Nucleus, 1997, Endocrinology 138: 2569-2576.)
Circulating thyroid hormone levels also fall in association with severe illnesses and infection (287), but use a different set of regulatory controls. This is based on the observation that both POMC and CART gene expression are increased in the arcuate nucleus (288) and circulating levels of leptin are elevated under these conditions (289). In addition, norepinephrine secretion is increased in the PVN, and ordinarily would be expected to stimulate the secretion of TRH (290). The precise anatomical pathways and mediators that override the activating effects of catecholamines, leptin and α-MSH on TRH neurons are not yet known. However, type 2 iodothyronine deiodinase (D2), an enzyme that converts thyroxine into the more biologically active thyroid hormone, tri-iodothyronine, is expressed in tanycytes and D2 expression and enzymatic activity is substantially increased by endotoxin (291). Given the location of tanycytes in the median eminence in contact with both the CSF and blood in the portal vascular plexus, and evidence that they express the thyroid hormone transporter, monocarboxylate transporter 8 (MCT8) (292), it is conceivable that tanycytes contribute to the fall in circulating thyroid hormone associated with infection by increasing the concentration of T3 in the mediobasal hypothalamus and suppressing the synthesis of TRH in hypophysiotropic neurons by local feedback regulation. Hence, tanycytes may extract T4 from the bloodstream or the CSF, convert T4 to T3, and then release T3 into the CSF that could diffuse into the PVN by volume transmission (293)after moving between ependymal cells lining the third ventricle; release T3 directly into the median eminence that could be taken up by TRH axon terminals and then transported retrogradely to the PVN; and/or concentrate in arcuate nucleus neurons that have known projections to TRH neurons in the PVN (278, 282, 283, 294). This mechanism is supported by observations demonstrating that the administration of LPS to D2 KO mice prevents the anticipated reduction in TRH mRNA observed in WT animals (295). T3 may also be released into the portal capillary system for conveyance to the anterior pituitary and contribute to the mechanism whereby endotoxin inhibits the secretion of TSH. The hypothesized regulatory mechanism is schematized in Fig. 34.
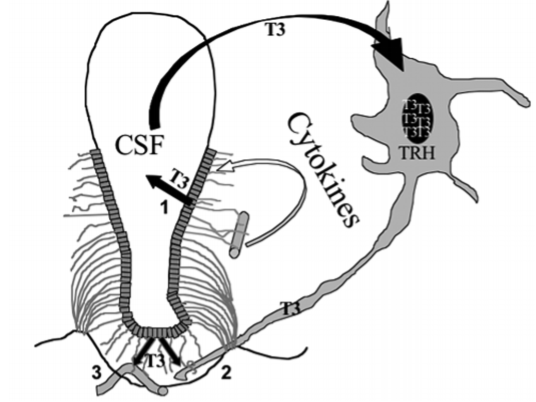
Fig. 34. Proposed mechanism for D2-regulation of the hypothalamic-pituitary-thyroid axis following the administration of LPS. LPS increases D2 activity in tanycytes resulting in increased T4 to T3 conversion. [1] T3 is released from tanycyte apical processes into the CSF for conveyance to the paraventricular nucleus, or [2] taken up from hypophysiotropic TRH axonal processes in the median eminence and transported retrogradely back to its cell body in the paraventricular nucleus. [3] T3 may also be released into the portal capillary system and directly inhibit the secretion of TSH. Local tissue hyperthyroidism inhibits TRH in the paraventricular nucleus. (From Lechan, R. M.; Fekete, C., Role of thyroid hormone deiodination in the hypothalamus. Thyroid 2005, 15, (8), 883-997.)
Cold exposure is another example of how afferent input to hypophysiotropic TRH neurons can alter the sensitivity of feedback regulation by circulating levels of thyroid hormone. In this instance, however, TRH secretion is increased (296). The mechanism involves catecholamine projection pathways from the brainstem to hypophysiotropic TRH neurons cell bodies and their axon terminals in the median eminence to increase both TRH mRNA and TRH release, respectively.
REGULATION OF GnRH SECRETION
Pulses of GnRH initiate the pulsatile release of anterior pituitary gonadotropins, and changes in the GnRH pulse frequency dictate how much LH and FSH ultimately will be released (297,298). This intermittent signal is of critical importance for pubertal development and necessary for the regulation and maintenance of normal reproductive function throughout the ovulatory cycle. In the absence of episodic GnRH release, such as with continuous, exogenous administration of GnRH, the synthesis and secretion of gonadotropins are profoundly suppressed as a result of desensitization of GnRH receptors (299). The central mechanisms governing the pulsatile secretion of GnRH may involve a variety of factors, but the most important would appear to be the recently discovered kisspeptin/G protein-coupled receptor 54 (GPR54) neuroregulatory system (300,301). The kisspeptins derive from a single precursor but comprise a group of peptide molecules ranging from 10-54 amino acids, all capable of binding and activating the G-protein coupled receptor GPR54 with similar efficacy (301,302). In humans, kisspeptin-54 has also been termed metastin on the basis that it was originally recognized to suppress cancer metastasis (303).
GnRH-producing neurons are located primarily in the preoptic region in rodents, but in all animal species give rise to axons that project caudally to terminate in the external zone of the median eminence (304). Although GnRH neurons may have intrinsic oscillatory characteristics that might explain the pulsatile secretion of GnRH (2305.306), evidence would support the importance of GPR54 and the kisspeptins in modulating their secretory responses and in particular, the resurgence of GnRH pulsatility during puberty. Namely, both humans and animals with GRP54 deficiency have hypogonadotropic hypogonadism despite normal development of GnRH neurons and normal LH and FSH secretion in response to GnRH (297,307), GPR54 mRNA is expressed by GnRH neurons (307), central and peripheral administration of kisspeptins potently induce gonadotropin secretion ( 308, 309), kisspeptin-induced LH secretion can be blocked with GnRH receptor antagonists (309,310), kisspeptin depolarizes ~90% of GnRH neurons in adult mice (311), and transgenic mice with targeted disruption of the Kiss1 gene (that gives rise to the kisspeptins) has an identical phenotype as transgenic mice deficient in GPR54 (312). As brief intravenous infusions of kisspeptin every hour for 48h induce pulsatile LH discharges similar to those observed during puberty, whereas continuous kisspeptin infusion in mice or monkeys downregulate LH secretion by desentensitizing GRP54 (313-316), it has been proposed kisspeptin-producing neurons comprise the pulse generator for GnRH neurons or at the very least, amplify the activity of the pulse generator (317).
Neurons producing the kisspeptins are located in the hypothalamic arcuate nucleus and in some species, the anteroventral paraventricular nucleus (AVPV) (316), and express the alpha estrogen receptor (318). Curiously, these two populations are regulated inversely to each other. Thus, kisspeptin gene expression in arcuate nucleus neurons increases following ovariectomy and decreases with estrogen administration, whereas the reverse occurs in anteroventral paraventricular cells (315,316). It has been proposed, therefore, that these two, kisspeptin neuronal populations may mediate the negative and positive feedback effects of estrogen on GnRH neurons (319), with the AVPV neurons involved in the estradiol/progesterone-induced preovulatory GnRH/LH surge (320). Functional heterogeneity among the two kisspeptin neuronal populations is exemplified by the observation that kisspeptin neurons in the AVPV are sexually dimorphic, being particularly prominent in females, but virtually absent in males secondary to increased testosterone levels in males during development, and explain the inability of male rodents to mount a LH surge (321,322). In addition, the arcuate nucleus population of kisspeptin neurons co-express neurokinin B and dynorphin (323), that contribute to the pulsatile release of GnRH through opposing actions on kisspeptin, neurokinin B stimulating and dynorphin inhibiting its secretion (324,325). For this reason, the population of kisspeptin neurons is commonly referred to as KNDy neurons. Phoenixin-20 amide (PNX), is also synthesized in kisspeptin neurons and may activate these neurons though an autocrine mechanism by binding to GPR173 receptors or through connections with other kisspeptin neurons (326). Arcuate nucleus kisspeptin neurons also mediate the inhibitory effects of anorexia on reproductive function via a leptin-dependent mechanism (327).
Kisspeptin-containing axon terminals have been observed to terminate on hypophysiotropic GnRH neurons, but only the minority of cells and with surprisingly few boutons (315,317). In contrast, kisspeptin-containing axons heavily inundate the median eminence and extensively intermingle with GnRH-containing axon terminals (315,317). Although axo-axonal interactions between kisspeptin- and GnRH-containing axon terminals have not been observed (315), these specializations may not be required for physiologic function in the median eminence. Evidence that peripheral administration of kisspeptin has a similar potent action on LH secretion as central administration (297), and physiologic data showing that exogenous administration of kisspeptin to hypothalamic explants deficient in GnRH neurons still potently release LH into the medium (313), strengthen the possibility that kisspeptin‘s actions may be directly on GnRH axon terminals in the median eminence. Figure 35 summarizes a hypothesized mechanism for the neuroendocrine regulation of GnRH neurons by kisspeptin.
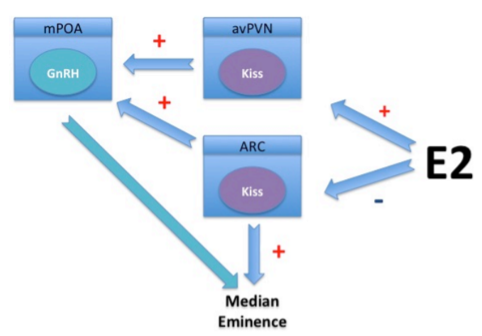
Figure 35. Simplified schematic demonstrating the mechanism for the regulation of GnRH secretion by kisspeptin. Two populations of kisspeptin neurons, oppositely regulated by estrogen, impinge on GnRH neurons in the medial preoptic nucleus that project to the neural-hemal contact zone in the median eminence. Kisspeptin-containing axon terminals may also interact with GnRH axon terminals in the median eminence.
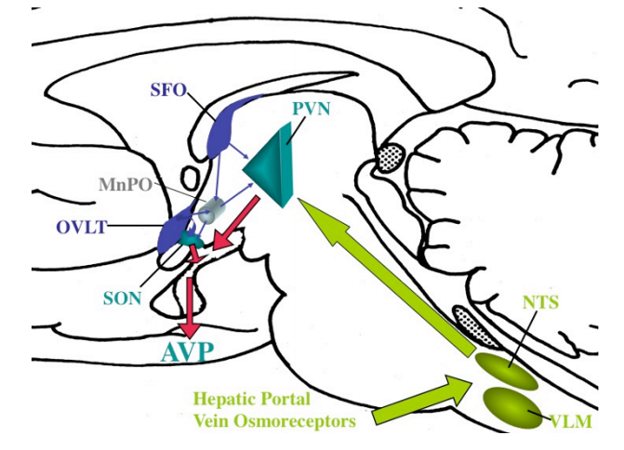
Glial-neuronal interactions in the median eminence and in contact with GnRH cell bodies may also be involved in regulating the delivery of GnRH to the portal system. In the median eminence, two mechanisms have been proposed (328-331). The first involves the release of glutamate, prostaglandins and growth factors from tanycytes that induce the secretion of GnRH from their axon terminals in the median eminence. The second involves plastic rearrangements between tanycyte end foot processes and GnRH axon terminals, allowing or disallowing secreted GnRH from entering portal capillaries. Thus, it is proposed during the preovulatory gonadotrophin surge, estrogen binds to alpha type estrogen receptors on tanycytes and induces tanycyte end feet retraction through PGE2-dependent production of TGFβ1, allowing GnRH axon terminals to establish better contact with the portal vessels (332). Estrogen may also affect the synthesis of adhesion molecules such as polysialylated neuronal cell adhesion molecule (PSA-N-CAM) and synaptic cell adhesion molecule (SynCAM) that facilitate glial-neuronal interactions and remodeling (328,329). The release of nitric oxide from portal vessel endothelial cells may also participate in tanycyte retraction by affecting actin cytoskeleton remodeling (332). Astrocytes have also been observed to enwrap GnRH perikarya and may similarly induce the release of GnRH through the release of PGE2 and/or TGFβ (331). It has also been hypothesized that astrocytes contribute to the mechanism whereby GnRH neurons are synchronized to generate the pulsatile release of the neuropeptide (331).
Mechanisms for reawakening of the reproductive axis during puberty is not fully understood, but appears to involve a decrease in transsynaptic inhibition to the GnRH neuronal system and increase in its stimulatory input from afferent neurons. GABAergic neurons are one of the major inhibitory inputs to the GnRH system and when inhibited, result in premature activation of the GnRH neuronal system. Increased stimulatory drive has been associated with glutamatergic transmission as well as increases in norepinephrine and NPY. Circulating levels of leptin also have an important role in the central modulation of puberty and reproductive function by food availability and nutritional status and can reverse the suppressive effects of undernutrition on the reproductive axis, but mediated primarily through kisspeptin neurons. Conversely, inhibition of reproductive function in association with stress appears to be mediated through CRH as suppression of gonadotropin secretion is reversible by administration of a CRH antagonist (333).
MODULATION OF PROLACTIN-REGULATING FACTORS
Prolactin secretion from the anterior pituitary is primarily under inhibitory regulation by dopamine neurons (A12 group of Dahlstrom and Fuxe) located in the arcuate nucleus. However, a number of prolactin-releasing factors have also been identified including TRH, oxytocin, VIP, vasopressin, histidine isoleucine, and serotonin, that can also trigger prolactin release under different physiologic circumstances following direct release into the portal system (334). For example, histidine isoleucine, which is co-expressed with CRH in parvocellular neurons in the PVN, and vasopressin are involved in stress-induced prolactin secretion through direct effects on lactotrophs. Serotonin has been implicated in lactation-induced prolactin secretion partly by stimulating TRH secretion from hypophysiotropic neurons, but also by inhibiting dopamine secretion and is further discussed below in the Lactation section.
Feedback control of prolactin secretion is mediated by a short-loop mechanism in which prolactin, itself, increases dopamine synthesis from the A12 neurons However, multiple neurotransmitter systems impinge on the A12 neurons that contribute to regulation of their neurosecretion including cholinergic neurons derived from the basal forebrain, hypothalamic glutamatergic and brainstem serotoninergic afferents that have activating effects and hypothalamic histaminergic and opiate peptide-containing afferents that have inhibitory effects (334).
Modulation of Vasopressin Secretion and Osmoregulation
Maintenance of the appropriate solute concentration in plasma (osmotic homeostasis) and plasma volume (volume homeostasis) is dependent upon two major factors, the perception of thirst and the ability to synthesize and secrete the antidiuretic hormone, arginine vasopressin from magnocellular neurons in the hypothalamic PVN (335). These two factors are closely interrelated such that amount of vasopressin circulating in the periphery is proportional to the plasma osmolality (336). Vasopressin induces cAMP and the translocation of specific aquaporin-2 water channels to the apical plasma membrane of tubular epithelial cells in the kidney, allowing water resorption (337,338). In addition, the rise in osmolality has independent behavioral effects. Thus, when plasma osmolality rises above basal levels, there is inducement to drink, shortly following the rise in vasopressin (335). While some vasopressin neurons in the PVN are intrinsically osmosensitive (339,340), the major mechanism of osmoregulation is via afferent pathways originating from osmoreceptor cells in other neuronal populations. These include inputs from the OVLT and the median preoptic nucleus, which if damaged, simultaneously abolish vasopressin secretion and thirst responses to hyperosmolality in both experimental animals and man (136,341). The SFO is also activated by a rise in osmolality and may contribute to vasopressin release through direct afferent projections to the PVN and/or to the OVLT using angiotensin II as a mediator (133-136,342). As mice with targeted disruption of the transient receptor potential (TRP) ion channels, TRPV1 and TRPV4, have impairment in vasopressin secretion and reduced drinking in response to hypertonic stimuli and show diminished cFos responses in the OVLT, these ion channels may be responsible for osmoreception (343). Along these lines, it particularly interesting that vasopressin can also be increased by hyperthermia (344,345), and that the trp genes are known to encode proteins involved in thermoregulation (see section E. Thermoregulation). Indeed, TRPV1 is required for thermosensory transduction of vasopressin secretion from isolated magnocellular neurons (346). Sodium channels may also contribute to the regulation of vasopressin secretion in response to hypernatremia by acting on neurons in the OVLT and SFO (347).
Whereas forebrain pathways communicate information about osmolality to the PVN, brainstem projections tend to carry nonosmotic, baroregulatory information, and important for vasopressin secretion, particularly in association with hypovolemia and hypotension (136,335). This information is carried through the vagus and glossopharyngeal nerves to the NTS and ventral lateral medulla, and then to the PVN through the ascending catecholaminergic pathways (Fig. 36). Magnocellular neurons in the PVN appear to be primarily innervated by the A1 catecholamine-producing cells in the ventral lateral medulla (247).
As described for hypothalamic tuberoinfundibular neurons, the threshold for vasopressin secretion by neurons of the magnocellular neurosecretory system can also be modified by their afferent signals as well as circulating factors. For example, the osmotic threshold for vasopressin release can be altered by glucocorticoids. Dexamethasone attenuates the vasopressin response to salt loading (348) and hypoadrenalism is commonly associated with the syndrome of inappropriate secretion of antidiuretic hormone (SIADH) that can be corrected by glucocorticoid administration (349). These effects are exerted directly on vasopressin neurons given the presence of glucocorticoid receptors in these cells (350). Other causes for SIADH, however, such as pulmonary disease and central nervous system disorders may be mediated through afferent pathways to the PVN. Hormone mediators of these projections include vasoactive intestinal polypeptide (VIP), acetylcholine, angiotensin II, neuropeptide Y and noradrenaline, among numerous others (351,352).
Appetite and Satiety
The demonstration that discrete regions of the hypothalamus control food intake was based on the early studies of Hetherington and Ranson (353) in 1940 showing that localized lesions of the hypothalamus result in obesity. These observations were seemingly confirmed in man when Reeves and Plum (354) reported that a discrete lesion (hypothalamic hamartoma) involving the hypothalamic ventromedial nucleus in a 28-year-old woman was associated with increased food consumption and profound obesity. In contrast, bilateral lesions of the lateral hypothalamus in animals result in anorexia and weight loss (353). Thus, the concept of a hypothalamic ventromedial nucleus satiety center and lateral hypothalamic orexigenic center that can be influenced by peripheral signals was developed, and dominated thinking about the hypothalamic control of feeding for several decades.
It was not until 1994, however, when the discovery of leptin revolutionized thinking on the mechanisms governing appetite and satiety (355). Leptin serves as an important humeral signal that reflects body fat stores, and by acting on discrete regions in the hypothalamus, orchestrates the behavioral, metabolic, and neuroendocrine adaptations to nutrient availability (285,286,356-359). Thus, during nutrient abundance, leptin secretion is increased, leading to decreased appetite and increased caloric disposal, whereas nutrient insufficiency leads to decreased leptin secretion, resulting in increased appetite, energy conservation, and a shift to a neuroendocrine profile that facilitates metabolic adaptation.
A major site of leptin’s action is the mediobasal hypothalamus, primarily the hypothalamic arcuate nucleus via specific receptors (Ob-Rb) that influence the activities of two separate groups of neurons with opposing functions through a number of signaling pathways that include Jak-STAT, PI3k-Akt-FoxO1, SHP2-ERK, AMPK, mTOR-S6K (360-362). These neurons include α-MSH-producing neurons that co-express CART, and AGRP neurons that co-express NPY (317). These neurons send monosynaptic projections to identical target regions within discrete regions of the hypothalamus where the signals are integrated and then relayed by independent pathways to regions of the brain governing feeding behavior, energy expenditure, and hypophysiotropic function (286,356-358). When circulating leptin levels are suppressed, such as during fasting, expression of genes encoding proteins that promote weight loss, and energy expenditure, α-MSH and CART, are reduced simultaneously with a marked increase in the genes encoding proteins that promote weight gain and reduce energy expenditure, AGRP and NPY. Cooperation between the opposing components of the regulatory system governing appetite and satiety is underscored by the biological action of AGRP as both a competitive antagonist and inverse agonist at melanocortin receptors (MC3r and MC4r) (285,363). Thus, during fasting, the rise in AGRP cooperates in down regulation of melanocortin signaling by antagonizing the action of α-MSH concurrently with inhibition of the POMC gene. Reciprocal connections between the arcuate nucleus NPY/AGRP neurons and α-MSH/CART neurons also are present (364), suggesting an even greater complexity to this regulatory system.
It is becoming increasingly recognized that the melanocortin signaling system may be the predominant regulatory system governing appetite and satiety. Whereas animals with targeted deficiency of NPY have an essentially normal phenotype and intact responses to fasting (365), animal models with targeted deletion of the type 4 melanocortin receptor (MC4r) and in humans bearing mutations that interfere with the function of the MC4r, the POMC gene, or the processing enzymes necessary to generate a fully mature α-MSH, develop a severe obesity syndrome (366-369). Loss of tone in the melanocortin signaling system as a result of senescence of the arcuate nucleus POMC neurons may also explain the tendency for weight gain with aging (370,371). Conversely, studies by Wisse et al (372) and Marks et al (373) have demonstrated that cancer cachexia can be prevented in experimental animals by the administration of melanocortin receptor antagonists. Maintaining adequate tone in the melanocortin signaling system, therefore, would appear to have an especially important role in the maintenance of normal body weight. Taking advantage of this observation is evidence that the MCRr agonist setmelanotide, has been shown to promote weight loss in individuals with leptin receptor or POMC deficiency (3740.
The arcuate nucleus is a main sensor of circulating levels of leptin, and by projecting to several different regions in the brain, provide the mechanism whereby leptin is capable of integrating a host of responses involved in energy homeostasis. The arcuate-PVN projection pathway has an important role in regulation of the thyroid axis (see above). Thus, hypophysiotropic TRH neurons in the medial parvocellular PVN receive direct projections from NPY/AGRP- and α-MSH/CART-producing neurons in the arcuate nucleus, altering the set point at which circulating thyroid hormone inhibits TRH (158,243,244,247) The end result during fasting is suppression of the HPT axis as a way to reduce energy expenditure. Other targets in the PVN include neurons in the anterior and ventral parvocellular subdivisions of the PVN on the basis that these neurons show phosphorylation of CREB following the central administration of α-MSH (375). Both subdivisions receive a high density of axons containing α-MSH and AGRP derived from the arcuate nucleus (376,377). Thus, these regions may be involved in some of the other actions of leptin including the regulation of feeding and/or energy disposal. This concept is supported by the observation that focal injections of α-MSH or α-MSH agonists directly into the PVN reduces feeding and can fully replicate the reduced feeding responses following icv administration (378). Conversely, α-MSH antagonists injected into the PVN have a potent effect to increase feeding (379). Since anterior parvocellular PVN neurons project to the limbic system (lateral septum and amygdala) (380,381), it is possible that this part of the PVN is involved in the behavioral manifestations of feeding. The ventral parvocellular subdivision is involved in the regulation of the autonomic nervous system through descending projections to brainstem and spinal cord targets (164,381,382). This region, therefore, may be involved in the regulation of energy disposal by controlling heat loss from brown adipose tissue through effects on uncoupling protein-1 (UCP-1) (383) and by affecting lipolysis and proteolysis in white fat and muscle, respectively (384). A population of POMC-responsive, glutamatergic PVN neurons descends to the parabrachial nucleus, now also considered to be an important brainstem nucleus involved in the suppression of appetite (385). Some parabrachial neurons project to the central nucleus of the amygdala using calcitonin gene-related transcript as a transmitter (386), implicating the central nucleus of the amygdala as another important node in the satiety pathway. Descending projections from leptin-responsive PVN neurons may also modulate the sensitivity of brainstem neurons to satiety signals originating from the gastrointestinal tract, mediated at the level of brainstem nuclei (387,388), and have been shown to affect the anorexigenic effects of peripherally administered CCK (389). Oxytocin may be one of several PVN-derived peptides relaying forebrain information about appetite and satiety to the brainstem (390).
In addition to the PVN, leptin-responsive neurons in the arcuate nucleus synapse on two separate populations of neurons in the lateral hypothalamus that produce melanin-concentrating hormone (MCH) and orexin (391). These neurons project to multiple regions of the brain including the cerebral cortex, midbrain and pons including the ventral tegmental area (VTA) and the nucleus accumbens, well-recognized reward centers involved in hedonic eating and addiction (392). MCH acts as an endogenous stimulator of food intake and its mRNA is increased during fasting (393,394) whereas orexin promotes arousal responses (395) that would have an essential role in permitting food-seeking behavior during periods of nutrient deficiency. Orexin also increases gastric contractility through projections to the brainstem, and by reducing gastric distension, suppresses satiety signals carried by the vagus nerve (396). Thus, during caloric restriction, activation of orexin- and MCH-producing neurons would have several actions to promote increased food ingestion and promote weight gain through effects on appetite, behavior and the incentive to feed. Leptin-responsive, CART-producing neurons in the arcuate nucleus also project directly to the intermediolateral cell column of the spinal cord (397), indicating their importance in autonomic control.
Leptin receptors are also expressed by other hypothalamic nuclear groups including the caudal part of the hypothalamic dorsomedial nucleus (DMN) and the dorsomedial division of the ventromedial nucleus (VMN) (398). Evidence that leptin-responsive regions of the brain other than the arcuate nucleus contribute to the regulation of feeding has been demonstrated by only a modest reduction in hyperphagia after the leptin receptor has been re-expressed selectively in arcuate nucleus of animals with leptin deficiency (399,400). The DMN has extensive projections to the PVN, particularly portions involved in autonomic control, as well as direct brainstem projections to the dorsomotor complex of the vagus (401). In addition, the DMN receives extensive projections from the arcuate nucleus, including projections from NPY/AGRP- and α-MSH/CART-leptin-responsive neurons (282,283). As lesions of the DMN produce hypophagia and reduce linear growth, this nucleus has an important role in the homeostatic control of feeding behavior (402). The DMN may also mediate the anorexic effect of cholecystokinin-8 (CCK-8) (403) through projections from CCK-8-producing neurons in the superior lateral subdivision of the parabrachial nucleus (404). The VMN has been long implicated in the regulation of feeding behavior as lesions of the VMN produce hyperphagia (405). However, these observations were likely due to transection of surrounding fiber pathways. Because the dorsomedial portion of the VMN projects to the subparaventricular zone, it has been proposed that leptin-sensitive VMN neurons may have a role in circadian rhythms (286).
The brainstem also contributes to leptin-regulated neuroendocrine responses involved in feeding, particularly the dorsal vagal complex (DVC) (406). The DVC is an important relay center in the brainstem for visceral sensory information carried by the vagus nerve from the liver GI tract, but also receives descending information from the forebrain including the PVN (356). Glucagon-like peptide (GLP-1)-producing neurons in the DVC have been shown to be leptin responsive (407), and this peptide has potent anorexic effects (408). NPY-producing neurons in the hypothalamic arcuate nucleus have been proposed as the primary target for GLP-1 due to the high concentration of GLP-1 receptors in this region and because GLP-1 can inhibit NPY-induced feeding (409,410). However, the arcuate nucleus contains relatively few GLP-1-containing nerve terminals as compared to the PVN and DMN (411), and discrete injections of GLP-1 into the PVN are capable of suppressing feeding (412). Leptin also enhances the anorexic effects of CCK (see below) that can be diminished by selective knock-out of leptin receptors in the DVC (413). Leptin also has important effects on dopaminergic neurons in the ventral tegmental area (VTA), that mediates motivated and reward-seeking behaviors including food consumption, as selective knock-out of the leptin receptor in this area results in increased feeding (414).
In addition to leptin, a number of gut peptides contribute to the central regulation of appetite and satiety (Table 10), either by acting directly on the hypothalamic arcuate nucleus or transmitted through the DVC via vagal and spinal afferent neurons (415-417). Among these are insulin, cholecystokinin (CCK), peptide YY (3-36), pancreatic polypeptide (PP), GLP-1, amylin, oxyntomodulin and ghrelin (418). Like leptin, levels of insulin vary with adiposity (316) and are suppressed by fasting and increased by eating. In addition, the intracerebroventricular administration of insulin reduces food intake and body weight (419) and prevents fasting-induced increases of NPY and AGRP mRNA in arcuate nucleus neurons (420). Amylin is co-released with insulin in response to a meal and functions as an anorectic hormone through effects on serotonin, dopamine and histamine (421). Both CCK and pYY are secreted by the gut in response to feeding and may have a role in the termination of eating, CCK through effects on receptors in visceral sensory axons that travel in the vagus nerve (422) and pYY through blood-borne inhibitory signals exerted through Y2 receptors on NPY/AGRP neurons in the hypothalamic arcuate nucleus (423). In addition to the central GLP-1 system described above, GLP-1 is also released into the circulation after a meal from the distal small intestine and colon, decreasing food intake through a vagal mechanism (424). Oxyntomodulin, which like GLP-1 derives from the same intestinal cells by posttranslational processing of proglucagon, binds to the GLP-1 receptor and has similar actions on reducing food intake that are abolished in the GLP-1 receptor knockout mouse (425). However, this peptide also appears to increase energy expenditure, raising the possibility of its particular utility in the treatment of obesity (426). As its name suggests, PP derives from the pancreas and inhibits food intake by binding to Y4 receptors in the DVC and arcuate nucleus and through effects mediated by the vagus nerve (427). Ghrelin, produced primarily by the stomach, is secreted directly into the bloodstream, but as opposed to all other identified gut-derived peptides, ghrelin stimulates food intake and is highest just prior to a meal and falls after eating (428). Thus, ghrelin may have a primary role in meal initiation. Its target is also the hypothalamic arcuate nucleus, increasing the expression of NPY/AGRP mRNAs (429) and inhibiting POMC neurons (430). However, vagotomy abolishes ghrelin-stimulated eating (431), indicating that ghrelin may also signal through the brainstem. Hypersecretion of ghrelin has been proposed as a mechanism for the morbid obesity associated with the Prader Willi syndrome (432). Nevertheless, treatment of Prader Willi patients with somatostatin analogues that suppresses circulating levels of ghrelin, does not improve the hyperphagia associated with this disorder (433), indicating that other mechanisms are responsible or that compensatory mechanisms take place. A second peptide derived from the ghrelin gene has been recently identified, obestatin, but its role in the regulation of appetite requires further characterization. Curiously, obestatin appears to suppress food intake (434). Nesfatin-1 has also been found to co-localize with ghrelin in gastric cells (435) and has potent satiety effects (436). While circulating nesfatin-1 can cross the blood-brain-barrier and inhibit NPY neurons in the arcuate nucleus (437), the peptide is highly expressed in the lateral hypothalamus in MCH neurons (438) and in the PVN and may be under the regulation of -MSH (436). New and potentially exciting chapters in gut-brain signaling relates to the importance of bile acids (439) and gut microbiota (417) in influencing central neural signaling primarily to affect energy homeostasis, but the mechanisms by which this takes place have yet to be fully elucidated.
Thyroid hormone should probably also be considered as a circulating hormone involved in appetite regulation through actions on the hypothalamus. Thyrotoxicosis is commonly associated with hyperphagia both in experimental animal models and humans (440-444), although the mechanism(s) by which this occurs are uncertain. Ishii et al (442) have shown an increase in hypothalamic NPY mRNA following the administration of thyrotoxic doses of T3, perhaps secondary to T3-induced increase in neuronal uncoupling protein 2 in NPY neurons (445). Nevertheless, T3-associated hyperphagia is only partially attenuated by a NPY receptor antagonist, indicating that other mechanisms must also be operable. The hypothalamus is richly endowed with thyroid hormone receptors (446,447), and nuclear T3 concentrations in hypothalamic extracts are elevated following the systemic administration of thyrotoxic doses of T3 or T4 (448). Recent studies by Kong et al (449) have demonstrated that the systemic administration of superphysiologic doses of T3 increase immediate early gene expression in the hypothalamic ventromedial nucleus. In addition, microinjection of T3 into the hypothalamic ventromedial nucleus induces a 4-fold increase in food intake during the first hour following injection. These data suggest a direct effect of T3 on the hypothalamus to induce feeding. Evidence that the nuclear T3 concentrations in the hypothalamus are elevated following the systemic administration of thyrotoxic doses of T3 or T4 (448) further substantiates this hypothesis. The coincidence that rats display a nocturnal pattern of feeding and that hypothalamic deiodinase activity follows a circadian pattern that increases during the night which may have the effect of increasing local tissue levels of T3 (444,449), provides circumstantial evidence for the potential importance of thyroid hormone to regulate feeding by increasing local, neural tissue concentrations of T3.
Estrogen also has an antiobesity effect and may contribute to the tendency for weight gain following menopause. The effects of estrogen appear to be mediated by the estrogen receptor-alpha (ERα), as mice with targeted deletion of this gene develop obesity (450). ERα is expressed by POMC neurons and when selectively ablated from these neurons, hyperphagia ensues (451).
Adipose-derived peptides (adipokines) other than leptin are also believed to have important roles in the regulation of appetite and energy expenditure (452). In particular, adiponectin serves as a starvation signal to preserve fat stores, increasing in the plasma with fasting. Adiponectin, thereby, functions inversely to leptin in the brain by binding to the adiponectin receptor 1 (AdipoR1) in the arcuate nucleus, and by activating AMPK, increases food intake and reduces energy expenditure by suppressing UCP-1 in brown adipose tissue (453).
Recent attention has also been given to the role of neurotransmitters and endocannabinoids in the regulation of food intake and energy expenditure (454,455). The cannabinoid receptor, type 1 (CB1) is the primary cannabinoid receptor in the central nervous system (456), and has a widespread distribution that includes the hypothalamus and limbic structures (457). Endogenous ligands for CB1, anandamide (AEA) and 2-arachidonoyl glycerol (2-AG), inhibit synaptic release of transmitters in both excitatory and inhibitory terminals (458). Since endocannabinoids are synthesized by neurons postsynaptic to axon terminals containing CB1 receptors, they are actually a retrograde signaling system. Neuronal release of endocannabinoids, therefore, regulates the activity of the cells’ own CB1-containing innervation. Endocannabinoids exert orexigenic effects when injected into the hypothalamus and may mediate the effects of ghrelin to increase food intake, block the inhibitory input to MCH neurons in the lateral hypothalamus and attenuate the excitatory input of anorexigenic neurons in the paraventricular nucleus (459,460). Endocannabinoids also activate the mesolimbic dopamine system that is involved in hedonistic eating (see below).
With respect to neurotransmitters, selective removal of the GABA transporter from AGRP neurons, for example, results in a lean phenotype, even on a high fat diet (461), whereas the selective deletion of leptin receptors from all neurons that express the GABA vesicular transporter develop marked hyperphagia (462). Serotonin is also known to have a profound effect on food intake, probably by acting on a subset of POMC neurons in the hypothalamic arcuate nucleus that express serotonin 2C receptors (463). Thus, two populations of POMC neurons have been proposed, those responsive to leptin that increase energy expenditure and those responsive to serotonin that reduce food intake (462, 464). These observations are in concert with the observation that serotonin agonists such as fenfluramine and lorcaserin have had efficacy in the treatment of obesity (465).
Heterogeneity of POMC neurons in the arcuate nucleus as defined by GABAergic and glutamatergic phenotypes has recently been recognized (466), although the physiologic significance is still uncertain. As noted above, dopamine has also been associated with reward-related food intake mediated through leptin-responsive, dopamine-producing neurons in the VTA (467). Dopamine has a major role in the regulation of reward processing including the desire for drugs of abuse and sweet foods (468). Recent evidence has linked impaired dopamine transmission in the mesolimbic nervous system to dietary obesity (469).
Nutrient sensing by the brain also appears to contribute to the regulation of appetite and satiety. Hypoglycemia increases food intake, presumably as a result of glucose deprivation on brainstem catecholamine neurons which project to the hypothalamus (470); the amino acid, L-leucine, but not other branched chain amino acids, exerts its anorexic actions by activating the mammalian target of rapamycin, mTORC1 pathway, in arcuate nucleus NPY/AGRP neurons by reducing NPY and AGRP (471) and in the caudomedial nucleus of the NTS (472); and fatty acids inhibit food intake by suppressing AMP-activated protein kinase (AMPK) in arcuate nucleus which similarly reduces NPY (473). Alpha-MSH-producing neurons in the arcuate nucleus are also excited by glucose-mediated closure of ATP-sensitive potassium (KATP) channels (474). The importance of this mechanism has been shown by Parton et al (475), demonstrating that expression of a mutant form of the KATP channel subunit Kir6.2b in α-MSH-producing neurons that impairs ATP-mediated closure of KATP channels, results in impaired glucose tolerance.
In addition to the major role of the hypothalamus and brainstem in the regulation of eating, many other regions of the brain are also involved, particularly in the human brain (476). As noted above, dopaminergic neurons in the ventral tegmental area and substantial nigra that project to the nucleus accumbens, striatum and orbitofrontal cortex are involved in hedonic feeding, leading individuals to seek highly rewarding, high caloric foods. Emotional eating may be mediated by projections from the amygdala to the hypothalamus to initiate a hunter response. Finally, cognitive control systems in the prefrontal cortex can allow for self-restraint, even though food may seem highly appealing.
A summary diagram of the complex mechanisms involved in the regulation of appetite and satiety is shown in Fig. 37.
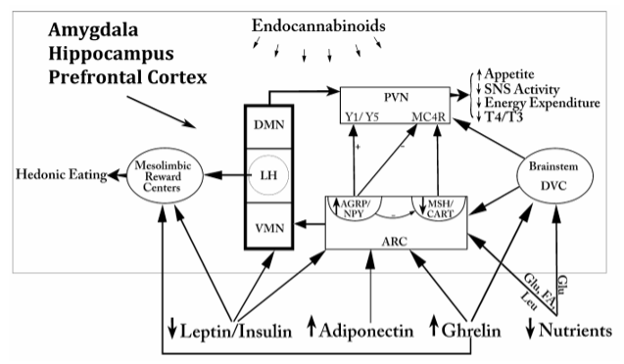
Figure 37. Simplified schematic representation of some of the regulatory factors and pathways involved in the regulation of appetite and satiety. Circulating factors derived from fat, pancreas, liver and the gastrointestinal tract converge on the hypothalamus and/or brainstem to orchestrate a series of responses that promote increased appetite, decreased energy expenditure, activation of mesolimbic reward centers and reduce circulating thyroid hormone levels. Note similarities of target regions in the brain by several regulatory factors, particularly for AGRP/NPY and -MSH/CART neurons in the hypothalamic arcuate nucleus. The endocannabinoid system exerts regulatory effects on neurons in multiple regions of the brain. Other regions of the brain including the amygdala, hippocampus, and prefrontal cortex also have important roles in the regulation of eating. ARC= arcuate nucleus, DMN= dorsomedial nucleus, DVC= dorsal vagal complex, LH= lateral hypothalamus, PVN= paraventricular nucleus, SNS=sympathetic nervous system, VMN= ventromedial nucleus.
Lactation
Suckling is a well-recognized physiological stimulus for increased prolactin secretion from the anterior pituitary gland and oxytocin from the magnocellular, neurohypophysial system (477). This stimulus is relayed to the hypothalamus via the spinal cord and brainstem (478,479), although the precise intrahypothalamic pathways involved have not been precisely elucidated (480). Using c-fos as a marker for neuronal activation, several potential relay centers in the brainstem have been identified including the ventrolateral medulla (A1 catecholamine cell group), locus coeruleus, lateral parabrachial nucleus, caudal portion of the paralemniscal nucleus, and lateral and ventrolateral portions of the caudal part of the periaqueductal gray (481). By inhibiting tuberoinfundibular dopamine neurons in the arcuate nucleus and increasing prolactin releasing factors in tuberoinfundibular neurons in the hypothalamic PVN and posterior pituitary (482), these signals permit high circulating levels of prolactin, particularly when dopamine has been inhibited. Putative prolactin releasing factors include thyrotropin-releasing hormone, oxytocin, serotonin, opioid peptides and TIP39, although the latter may function by increasing dynorphin (480). Prolactin also feeds back on dopamine neurons in the arcuate nucleus to inhibit the release of dopamine (483), but this ultrashort feedback loop is inhibited during lactation by dissociation of electrical activity and dopamine release from dopamine neurons, allowing for sustained elevations in prolactin in response to suckling (484). Prolactin is not only important for the synthesis and maintenance of milk secretion, but contributes to the physiologic responses that complement lactation including the development of material behavior and inhibition of reproductive function (477). These effects are facilitated by upregulation of prolactin receptors in the choroid plexus during lactation (485), allowing increased entry of prolactin from the circulation into the brain through a carrier-mediated transport system, as well as in several hypothalamic nuclear groups including the medial preoptic nucleus, PVN, supraoptic nucleus and arcuate nucleus (486). In particular, binding of prolactin to its receptor in the medial preoptic nucleus may be important for maternal behavior, as antagonizing prolactin receptors with a specific prolactin receptor antagonist injected directly into the preoptic area delays the onset of material behavior (487). In addition, inhibition of pulsatile gonadotropin secretion associated with lactation may be at least partly mediated by the effects of prolactin on GnRH neurons in this region (477), although recent evidence for suckling-induced inhibition of kisspeptin gene expression in arcuate nucleus neurons may also be contributory (488.489).
Suckling also results in a marked increase in NPY gene expression in arcuate nucleus neurons (490,491), mediated both by neuronal afferents relayed from the brainstem and by the fall in circulating levels of leptin brought about by negative energy balance resulting from milk production (492). As described above (see C. Appetite and Satiety), NPY is a powerful orexigenic substance. Its increase during suckling, therefore, has been proposed to explain the hyperphagia associated with lactation to meet the energy demands imposed by milk production (492), although other changes are also observed in arcuate nucleus neurons that may contribute to hyperphagic responses including an increase in AGRP gene expression and decrease in POMC and CART mRNAs (493,494), similar to that described above for fasting. NPY-containing axon terminals of arcuate nucleus origin also richly innervate the medial preoptic region and can be found in close proximity to GnRH neurons (495). The suckling-induced rise in NPY, therefore, may also contribute to inhibition of reproductive function (494) through direct actions on GnRH neurons by binding to NPY Y5 receptors (496).
The other major component of the suckling response is the effect on oxytocin release from the posterior pituitary to allow milk ejection. While some of the anatomical pathways responsible for this response may parallel with those inducing prolactin secretion, distinct pathways are also likely to exist. Particularly remarkable about this mechanism is that during suckling, oxytocin neurons on both sides of the hypothalamus in the paraventricular and supraoptic nucleus are synchronized to release oxytocin in a pulsatile manner throughout the suckling stimulus. This coordinated pattern of release may be regulated by afferent inputs to the oxytocin neurons that include glutamatergic neurons arising in the lateral septum and bed nucleus of the stria terminalis (480). Anatomic plasticity of the oxytocin neurosecretory system also contributes through reraction of astoglial processes that normally separate oxytocin neurons, allowing for increased somatic appositions (497). Local release of oxytocin from somata and dendrites (intranuclear release) in response to suckling may also contribute by modulating the effect of neurotransmitters, increasing oxytocin gene expression and through local effects on astroglia to promote somatic appositions (480).
The circuitry involved in the coordinated responses to suckling is illustrated in Fig. 38.
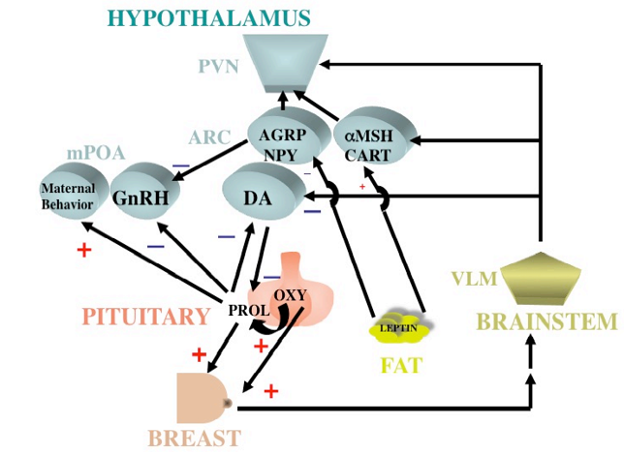
Figure 38. Schematic drawing of the major pathways involved in lactation. Suckling leads to neurogenic responses mediated through the medulla to inhibit dopamine secretion in arcuate nucleus (ARC) neurons and stimulate oxytocin (OXY) secretion in paraventricular (PVN) neurons. Prolactin and oxytocin exert effects on the breast, but prolactin also gains entry into the CNS to affect maternal behavior and inhibit reproductive function by acting on medial preoptic neurons (mPOA), and further inhibit the secretion of dopamine from ARC neurons. Milk production leads to a fall in circulating levels of leptin causing an increase in NPY and AGRP and inhibition of POMC in ARC neurons. NPY is also increased by neurogenic signals from the brainstem. Inhibitory effects of NPY on GnRH-producing neurons in the mPOA contributes to inhibition of reproductive function. NPY also exerts direct effects on the PVN to induce increase feeding and promote energy conservation.
Thermoregulation
The hypothalamus is the primary locus for coordinating thermoregulatory information and integrating thermoregulatory responses (498-500). It continually monitors local brain temperature through temperature sensitive neurons and by utilizing thermoreceptors in the skin, abdominal cavity and spinal cord, and then orchestrates a series of responses to maintain normal, core body temperature by utilizing the autonomic nervous system, altering behavior, and through neuroendocrine responses. Thyroid hormone is a necessary component for heat regulation since in its absence (myxedema), hypothermia commonly develops.
Although thermosensitive neurons can be found throughout the hypothalamus (401), the most important thermoregulatory locus is the preoptic region including neurons in medial and lateral portions of the preoptic nucleus, anterior hypothalamus including the perifornical region, dorsomedial nucleus and nearby regions of the septum. Although most of the data on the anatomy of thermogenesis arises from work in rodents, evidence that the preoptic/anterior hypothamus is also the critical hypothalamic area for regulating thermogenesis in humans is apparent by functional MRI (502). Preoptic cooling increases heat production by inducing shivering, or by nonshivering thermogenesis mediated by sympathetic activation of uncoupling protein-1 (UCP-1) in brown adipose tissue that allows mitochondria to generate heat from ATP, and by increasing intermediary metabolism in muscle and other parenchymal organs. In addition, cooling induces heat retention responses by cutaneous vasoconstriction and redirecting blood flow from cutaneous to deep vascular beds, results in behavioral responses (seek warmer environment, put on more cloths, increase food intake), and in some animal species, increases thyroid thermogenesis by activating the hypothalamic-pituitary-thyroid axis (503). Conversely, preoptic warming reduces heat production and increases heat loss responses through vasoconstriction, sweating, increased respiration (panting), inhibition of UCP-1 in brown adipose tissue, and specific behavioral responses (501).
The recent discovery of substantial amounts of brown adipose tissue are also present in adult humans (504,505) has indicated that like smaller mammals, brown adipose tissue has a similar role in whole body thermogenesis in man. Just a few grams of brown adipose tissue may be adequate to increase daily energy expenditure in humans by ~20% (506). Indeed, knowledge of the regulatory circuits and factors that govern thermogenesis in brown adipose tissue (see above section on Appetite and Satiety) has provided insight for the development of innovative molecular and pharmacological approaches to achieve weight loss (507), although still at a preliminary stage.
Two types of thermosensitive neurons can be found in the preoptic region, warm sensitive neurons that increase their firing rate when preoptic temperature rises and cold sensitive neurons that increase their firing when preoptic temperature falls (501). However, the warm sensitive neurons predominate both in cell number and in importance of the regulatory responses for both heat loss and heat production mechanisms. Thus, lesions involving the preoptic region per se are commonly characterized by abnormalities in heat dissipation and lead to hyperthermia and elevated temperature in brown adipose tissue (502). Surprisingly, the neurotransmitter/peptide mediators and pathways mediating thermoregulatory responses are not precisely known. When injected directly into the preoptic area, however, a number of different substances can induce hypothermic or hyperthermic responses (Table 11). Since thermoregulation involves the coordination of multiple responses that can differ between animal species (i.e., panting in the dog, increased salivation in the rat which can be applied to the fur to enhance evaporative heat loss, sweating in man), it is logical that several different pathways are utilized. Evidence suggests that efferent pathways governing shivering involve ipsilateral and crossed fibers (508) that traverse the median forebrain bundle to terminate in the posterior hypothalamus, using GABA as a neurotransmitter (509). The pathway continues caudally through the midbrain, dorsolateral to the red nucleus, and interacts with reticulospinal neurons. It then proceeds through the reticulospinal tract, to innervate α-motor neurons in the ventral horn of the spinal cord. Regulation of heat production in brown adipose tissue also proceeds from preoptic neurons through the medial forebrain bundle to hypothalamic nuclear groups involved in autonomic regulation, particularly the PVN, FiDMN and VMN (509). The PVN has direct efferent projections to preganglionic neurons in the intermediolateral column of the spinal cord which give rise to the sympathetic innervation of brown adipose tissue (510). Regulation of cutaneous blood flow also proceeds from thermosensitive neurons in the preoptic region through axons descending in the medial forebrain bundle, but likely relayed to neurons in ventrolateral portions of the midbrain periaqueductal gray (PAG) before proceeding to sympathetic preganglionic neurons in the spinal cord. PAG neurons show strong c-fos induction following unilateral preoptic region heating (511) and induce cutaneous vasodilatation when stimulated (512). Preoptic warming also inhibits vasoconstrictor neurons in the medullary raphe (raphe magnus and pallidus) (513) that have projections directly to preganglionic sympathetic neurons in the intermediolateral column of the spinal cord (514). Pathways mediating behavioral changes associated with thermoregulation are unknown.
Table 11. Substances That Exert Thermoregulatory Effects in the CNS
|
HYPOTHERMIC
|
HYPERTHERMIC
|
ACETYLCHOLINE
ANGIOTENSIN II
CCK
DOPAMINE
ESTROGEN
α-MSH
NEUROTENSIN
NOREPINEPHRINE
OPIOID PEPTIDES
SOMATOSTATIN
SUBSTANCE P
VASOPRESSIN
|
CRH
GABA
OPIOID PEPTIDES
PROGESTERONE
PROSTAGLANDINS
SEROTONIN
TRH
|
While it is clear that the transient receptor potential ion channels, including TRP vanilloid 1 (TRPV1), TRPV3, TRPV4, and TRPV8, are involved as heat receptors in the periphery (515), it remains controversial whether these receptors contribute to thermoregulatory responses at the level of the preoptic nucleus (516). TRPV1 mRNA is present in the hypothalamus, and administration of capsaicin, a ligand for TRPV1, directly into the preoptic hypothalamus induces hypothermic responses, suggesting activation of warm-sensitive neurons (517,518). Nevertheless, mice deficient in TRPV1 show normal thermoregulation when placed in a warm environment (519), raising questions about the physiologic importance of TRPV1 in central thermoregulatory responses. Recently, however, melastatin type 2 ion channels (TRPM2) have been identified in the preoptic nucleus that when selectively activated or inhibited results in profound hypothermia or hyperthermia, respectively (520). In addition, transgenic animals deficient in TRPM2 have an exaggerated fever response.
Under normal circumstances, there is a diurnal variation of body temperature, highest in late afternoon and early evening and lowest in morning upon arising. The hypothalamic suprachiasmatic nucleus controls this rhythm, and would appear to do so through direct projections to the dorsal portion of the subparaventricular zone, a region just ventral to the PVN (see F. Circadian Rhythmicity). Thus, bilateral focal lesions in the dorsal subparaventricular zone disrupts the circadian variation of body temperature, whereas bilateral lesions of the PVN, itself, is without effect (521). Since the subparaventricular zone has prominent projections to the preoptic area (522), it is presumed that the suprachiasmatic nucleus relays information to thermosensitive neurons in the preoptic region by a multisynaptic pathway involving the subparaventricular zone (521). However, the precise targets in the preoptic region from subparaventricular zone neurons have not yet been identified.
The set point for temperature regulation is also sensitive to circulating levels of sex steroids, with core body temperature falling just prior to the midcycle surge in women and rising during the luteal phase (523,524). Estrogen, itself, would appear to be responsible for the fall in temperature in the late follicular phase by increasing the firing rate of preoptic warm sensitive neurons (525), whereas progesterone increases temperature in the luteal phase by decreasing the firing rate of preoptic warm sensitive neurons and, perhaps, increasing the firing rate of cold sensitive neurons (526). Both steroids readily pass the blood-brain barrier and thereby are presumed to act directly on thermosensitive neurons in the preoptic nucleus (527). Not unexpectedly, therefore, the lack of estrogen in the postmenopausal period gives rise to altered thermoregulatory responses (hot flashes) that occurs in over 80% of this group, and can be readily reversed by the administration of estrogen. It has been proposed that in the absence of estrogen, the sensitivity of warm sensitive neurons to even small increases in body temperature are increased (528). Along these lines, it is of interest that in one study, the frequency of hot flashes was greatest during the afternoon and evening when body temperature normally rises under the influence of the suprachiasmatic nucleus circadian pacemaker (529). The therapeutic response of some women with postmenopausal hot flashes to clonidine (530), an α2-adrenergic agonist, would indicate that catecholamines also participate in the heat loss responses. Recent evidence, however, points to neurokinin B derived from kisspeptin (KNDy) neurons as having an important role. Kisspeptin neurons project to thermosensitive preoptic neurons that express neurokinin 3 receptors (NK3R), and when a NK3R agonist is administered into the proeptic area or neurokinin administered peripherally to human subjects, heat dissipation responses are observed (531,532). Conversely, administration of an oral NK3R antagonist to postmenopausal women reduces the frequency and severity of hot flashes (533, 534).
A number of other substances have also been shown to modulate thermoregulatory responses, with thyroid hormone being one of the best studied examples and eluded to above. Thyroid hormone is essential to sustain the effect of sympathetic activation of brown adipose tissue to produce heat. Norepinephrine increases type 2 iodothyronine deiodinase in brown adipose tissue, which by converting T4 to T3 increases UCP-1 in mitochondria to convert stored energy into heat. Although best known for its effects on arousal, orexin-A also is involved in the regulation of body temperature through effects on brown adipose tissue thermogenesis by increasing sympathetic activity and may explain dysregulation of thermogenesis in individuals with narcolepsy (535). A role for prostaglandins in the febrile response associated with infection is discussed below.
Under certain circumstances, there is an adaptive advantage to elevate body temperature beyond the normal physiologic range that is highly conserved among animal species. Such is the situation during infection, when fever is a necessary response to facilitate recovery by improving the efficiency of immune cells and impairing replication of microorganisms (536,537). This homeostatic response is achieved by altering the thermoregulatory set point in medial preoptic neurons, but through a different mechanism than described above. Under these circumstances, it is proposed that circulating endotoxin and proinflammatory cytokines interact with specific receptors on vascular endothelial cells and/or subendothelial microglia in the OVLT, resulting in activation of cyclooxygenase and production of PGE2 (538.539). PGE2 released into the surrounding tissue binds to neighboring warm sensitive neurons in the median preoptic nucleus that express EP3 prostaglandin receptors (540), which by reducing GABAergic inhibition of thermogenic neurons in the hypothalamic paraventricular and dorsomedial nuclei and/or brainstem rostral ventromedial medulla (rMR, comprised of serotonergic neurons in the raphe pallidus and magnus, sympathetic premotor neurons), influence sympathetic preganglionic neurons in the spinal intermediolateral cell column that contribute to the generation of fever, cutaneous vasomotion, tachycardia and shivering (515, 541, 542). The proposed mechanism is schematized in Fig 39.
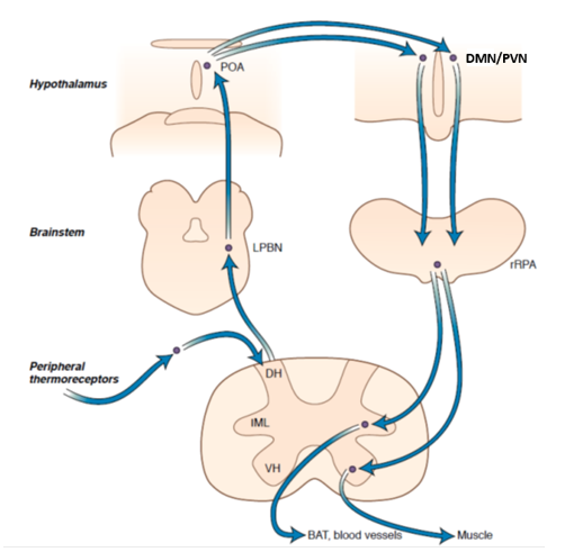
Figure 39. Simplified diagram of major loci and pathways of the temperature control center. DH=dorsal horn, DMV=dorsomedial hypothalamus, IML=intermediolateral cell column, LPBN=lateral parabrachial nucleus, POA=preoptic area, PVN=paraventricular nucleus, rRPa=rostral raphe pallidus, VH=ventral horn. (Adapted from Roth J and Blasties CM, Mechanisms of fever production and lysis: Lessons from experimental LPS fever, Comp Physio 2014;4:1563-1604, 2014 and Lechan RM, Neuroendocrinology, in Williams Textbook of Endocrinology, 14th Edition, Hypothalamus and Pituitary, Ch 7, S. Melmed, R Auch us, A gold fine, RJ Koenig, C Fose, Eds, Elsevier, 2019.)
Blasties et al (543) suggest an alternative mechanism for fever induction in which PGE2 release into the preoptic region is mediated by norepinephrine, arising in noradrenergic (A2 cell group) neurons in the ventrolateral medulla. This is based on the observation that in guinea pigs, the intra-preoptic microdialysis of α2-receptor antagonists potentiate the febrile response to LPS (544). The mechanism proposed involves activation of hepatic branches of the vagus nerve by mediators (possibly PGE2) produced by liver Kupffer cells following the systemic administration of LPS. Vagal afferent signals are then carried to the nucleus tractus solitarius in the brainstem, and after projecting to noradrenergic neurons in the ventrolateral medulla (A2), ascend in the ventral noradrenergic pathway and medial forebrain bundle to terminate in the preoptic region. Ek et al (544) have also demonstrated in the rat that the intravenous administration of interleukin-1 is capable of activating vagal sensory neurons in the nodose ganglion and can be attenuated by inhibitors of prostaglandin synthesis.
In addition to inducing fever, endotoxin simultaneously activates an endogenous, counterregulatory, antipyretic response, to prevent body temperature from rising too severely. This is largely achieved by stimulating the hypothalamic-pituitary-adrenal axis (see above) that exerts a dampening effect on the cytokine response, but more specifically by the direct antipyretic actions of α-MSH within the CNS (545). The latter situation occurs only in association with cytokine activation, as α-MSH has no effect on temperature regulation in the absence of fever (545,546). Alpha-MSH arises from the neuronal population in the hypothalamic arcuate nucleus (288), and while it is unknown precisely where α-MSH exerts its actions, the preoptic region including the VMPO is heavily innervated by axon terminals containing -MSH, suggesting a direct effect on thermosensitive neurons (547). Alpha-MSH is also contained in axons that heavily innervate autonomic regulatory neurons in the parvocellular PVN and the hypothalamic DMN, providing an alternative route for regulatory control over vasomotor responses and heat generation.
Circadian Rhythmicity
Circadian rhythms are genetically determined, cyclic modifications of specific physiological functions and behaviors, generated through endogenous mechanisms in nearly all living organisms (548,549). The basic organization of the circadian timing system includes an endogenous rhythm generator or pacemaker (also called endogenous clock or zeitgeber), a light-dark receptive system to entrain the endogenous clock to the time of day mediated by retinal photoreceptors (mainly cones) and visual pathways (retinohypothalamic pathway), and an efferent neural system coupling the pacemaker activity with effector systems in the brain that give rise to specific physiological functions and behaviors (548,549). The master clock in mammals is the hypothalamic suprachiasmatic nucleus (SCN), a small, paired nucleus embedded in the dorsal surface of the optic chiasm. Contained within this nucleus are multiple, small neurons that produce autonomous, self-sustaining oscillations synchronously firing to generate a common rhythmic output, perhaps mediated by the local release of GABA (550,551). If the SCN is lesioned bilaterally, “free-running circadian rhythmicity” is produced, characterized by disruption of the sleep-wake cycle and loss of predictable daily oscillations in feeding, drinking, melatonin secretion and the secretion of some anterior pituitary hormones (552,553). Normal rhythmicity can be restored if the SCN is transplanted back into the lesioned animals (554).
Molecular mechanisms for the endogenous pacemaker activity of SCN neurons have been attributed to clock genes that include period (per), Clock, Cryptochrome (Cry), and Bmal (548,549). Circadian oscillations in a number of other gene products mediated by microRNA (miR) have also been described in other tissues such as the liver (miR-122), retina (miR-26) and brain circuitries involved in locomotion (miR279) without any requirement for miRNA rhythmicity. Presumably this mechanism involves translational regulation of clock protein mRNA by miR accumulation, and/or functional heterogeneity of miRNA species in a single, constitutively expressed miR population (555). In addition, beyond transcriptional-translational feed-back loops occurring intracellularly, increasing evidence suggests that timekeeping mechanisms can be also controlled through non-transcriptional oscillators (NTO). Examples of NTO in mammals are the redox based rhythms in post-translational modification of the antioxidant peroxiredoxin (PRX) proteins found in mouse liver and human red blood cells, suggesting that NTO can be coupled to classical transcriptional-translational feedback loops to regulate circadian rhythmicity (556).
Two different subdivisions of the SCN have been described, a ventrolateral and dorsomedial subdivision (548). The ventrolateral subdivision or “core”, receives the major input to the SCN, including a massive projection of pituitary adenyl cyclase-activating peptide (PACAP)- and nitric oxide (NO)-containing axons from the retinohypothalamic pathway, GABA- and NPY-containing axonal projections from the intergeniculate leaflet of the thalamus, and serotonin neurons from the midbrain raphe (548,549). These inputs have an important role in modulating the endogenous rhythms of the individual SCN pacemaker cells during the day / night alternance and as a result of changes in locomotor activity (557). The dorsomedial subdivision or “shell”, primarily serves as the field for afferent information coming from the limbic system (hippocampus, bed nucleus of the stria terminalis, septum) and the hypothalamus, itself. It is likely that through these inputs, cognitive and emotional information may exert phase-shifting effects on SCN pacemaker activity (528). Both subdivisions are composed of a heterogeneous population of immunocytochemically distinct neurons. The ventrolateral SCN contains neurons that express vasoactive intestinal polypeptide (VIP), gastric-releasing peptide and GABA (558). The VIP-containing population seems to play a role in coordinating the different SCN neuronal groups involved in entraining different cyclic activities, thus ensuring intra-SCN synchrony (548). Dorsomedial neurons express arginine vasopressin (AVP), angiotensin II, somatostatin, GABA (548) and prokineticin 2 (PK2) (559). PK2 neurons have a distribution similar to AVP cells, and would be active during the light phase to favor locomotor patterns and thermoregulation, whereas they would remain silent at night (560). However, while ventrolateral subdivision neurons receive light information, most of these neurons do not produce rhythmic patterns (561). In contrast, the dorsomedial subdivision does contain rhythmic neurons, particularly apparent for AVP-producing neurons in which the peptide peaks during the day and is lowest at night (562). This rhythmic pattern is partly secondary to the presence of binding sites for clock genes in the AVP promoter region (563), but also dependent upon synaptic transmission from other SCN neurons (564), perhaps those in the ventrolateral subdivision through intra-SCN connections (565) where VIP-containing cells are the best candidates (559).
The SCN has massive projections to three major regions of the diencephalon. The most important is the hypothalamic subparaventricular zone (SPVZ). Projections from the dorsal SPVZ reach the medial preoptic hypothalamus and are involved in the regulation of body temperature set-point and food-dependent energy intake (558). In contrast, projections from the ventral SPVZ heavily innervate the hypothalamic dorsomedial nucleus and, to lesser extent, the midline thalamus, midbrain reticular formation and basal forebrain. These outputs entrain photic stimuli with changes in food intake, rest/locomotion behavior, sleep-wake phases and pituitary hormone secretions. The second major projection is a GABAergic fiber tract to the hypothalamic PVN, and is primarily related to the secretion of melatonin from the pineal gland (558,566,567) by way of a multisynaptic pathway involving dorsal parvocellular neurons in the PVN, preganglionic cholinergic neurons in the intermediolateral cell column of the spinal cord, and postganglionic noradrenergic neurons in the superior cervical ganglion (567,568). Melatonin is of importance as a humoral signal that feeds back on the SCN through melatonin receptors expressed in this nucleus, to facilitate sleep onset by communicating information concerning initiation and length of the dark phase (450,452). In addition, it regulates immune function, is of particular importance for reproductive activity in animals with seasonal breeding patterns (569,570), and participates in accommodating pituitary function with the shift to torpor in hibernators (571,572). In homeothermic mammals, melatonin has been implicated in the inhibition of pituitary TSH biosynthesis at night (559). A direct projection to the PVN may also mediate the day / night shift of anterior pituitary hormone secretion. In rats, the SCN inhibits hypophysiotrophic CRH release through excitatory AVP outputs impinging onto GABAergic PVN interneurons. In contrast, through direct SCN AVP outputs to tuberoinfundibular CRH cells (and possibly glutammateric inputs), release of CRH is triggered just before awaking, establishing circadian oscillation in the ACTH-glucocorticoid axis. At the same time, autonomic PVN neurons are activated to stimulate the downstream, spinal, sympathetic outflow to the adrenal gland, resulting in increased ACTH sensitivity of the zona fasciculata (573,574). However, circadian oscillations in CRH, ACTH and glucocorticoid release can also be regulated by the endogenous clock genes, per1, per2 and Bmal1, that exhibit antiphasic patterns in the PVN and adrenal cortex with respect to the pituitary corticotrophes. The expression of these three, clock genes, in fact, can be differentially influenced by restricted feeding, acting as a metabolic effector independent of SCN outputs (575). Similar to the sympathetic projections to the adrenal, the thyroid gland is also believed to receive an indirect SCN input via PVN neurons, activating sympathetic spinal outflow that would contribute to circadian regulation of thyroid hormone secretion (559). A third major projection is directed to the medial and lateral tuberal hypothalamus, primarily the ventromedial nucleus, arcuate nucleus and lateral hypothalamic area. These fibers are believed to influence the regulation of the neuroendocrine tuberoinfundibular and neurohypophysial secretions. Figure 40 schematically depicts an integrated view of the mammalian circadian timing system and the main physiological functions and behaviors it controls.
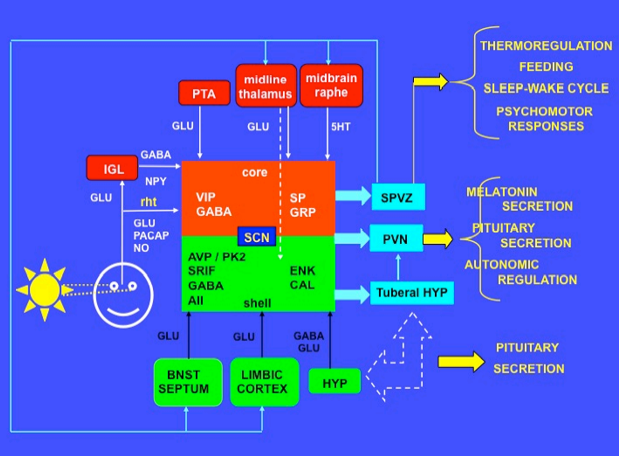
Figure 40. Schematic drawing showing an integrated view of the mammalian circadian timing system and the main neuroendocrine responses, physiological functions and behaviors under its control. AII = angiotensin II; APv = anterior periventricular nucleus of the hypothalamus; AVP = arginine vasopressin; BNST = bed nucleus of the stria terminalis; CAL = calretinin; ENK = enkephalin; GABA = aminobutyric acid; GRP = gastrin-releasing peptide; GLU = glutamic acid; 5-HT = 5-hydroxytryptamine or serotonin; HYP = hypothalamus, IGL = intergeniculate leaflet; NO = nitric oxide; NPY = neuropeptide Y; PACAP = pituitary adenylyl cyclase-activating peptide; PK2= prokineticin 2; PTA = pretectal area; PVN = hypothalamic paraventricular nucleus; rht = retinohypothalamic tract; SCN = suprachiasmatic nucleus; SP = substance P; SPVZ = hypothalamic subparaventricular zone; SRIF = somatostatin; VIP = vasoactive intestinal polypeptide.
Sleep-Wake Cycle
Sleep is a natural state of altered consciousness, easily reversible, self-regulating and characterized by a stereotypic posture, decrease in voluntary motor activity and increase in arousal threshold. In mammals and man, sleep periods are cyclically coupled to periods of wakefulness, giving rise to a circadian sleep-wake cycle. Electrophysiologically, sleep is characterized by a progressively slower, higher voltage, and more synchronized electrical activity of the cortex (alpha waves – stage 1, spindle and k-complexes – stage 2, delta waves – stages 3 and 4) as opposed to wakefulness where fast, low voltage, and desynchronized electrical activity prevails (beta waves). Only relatively brief times are spent in sleep-wake transitions. Episodes of partial arousal without wakefulness occur during sleep, and are characterized by desynchronized electrical cortical activity resembling the EEG pattern of wakefulness and the initial sleep phase (theta waves). This arousal is coupled to rapid eye movements (REM) and loss of muscle tone (except for respiratory and inner ear muscles). In contrast, non-REM or NREM sleep is devoid of involuntary eye movements and muscle tone resumes, leading to deep sleep (576).
The basic neural organization of the sleep-wake cycle relies on two adjacent areas of the neuraxis, the diencephalon-basal forebrain and the mid-rostral brainstem, collectively expressing two, stable, firing states to produce either rest or arousal, with a tendency to avoid intermediate conditions (flip-flop switch). In this manner, inappropriate behavioral fluctuations that might endanger survival are avoided, favoring discrete and rapid changes between sleep and waking profiles (or between REM and NREM sleep) above a background of slow and continuous variations in circadian and homeostatic inputs (either photic or non-photic autonomic, endocrine-metabolic and immune stimuli) (576,577).
The neural structures participating in this flip-flop switch establish reciprocal, feedback circuitries and can be classified as sleep- and wakefulness-promoting centers, the latter including specific cell groups that trigger and shut off the REM arousal state during sleep. The primary sleep center is localized in the preoptic hypothalamus, and involves GABA- and galanin-containing neurons in the ventrolateral preoptic nucleus or VLPO (578). A secondary sleep center is located in the midline thalamus (visceral or limbic thalamus), primarily in the dorsomedial (579) and reticular (580) thalamic nuclei.
Wakefulness centers are numerous and in large part belong to the ascending reticular activating system of Moruzzi and Magoun (581). This system can be subdivided in two different components, including monoaminergic and cholinergic cell groups in the pontine and mesencephalic (or limbic midbrain area) reticular formation. The monoaminergic neurons comprise the noradrenergic locus coeruleus (LC), the serotoninergic median and dorsal raphe (DR) and parabrachial nucleus (PBN) (including some neurons that co-contain glutamage), and the dopaminergic ventral periaqueductal grey (vPAG) in the tegmentum of the mesencephalon. The cholinergic neurons are located in the pontine tegmentum, specifically in the laterodorsal (LDT) and peduncolopontine tegmental (PPT) nuclei, respectively. In addition to pontine and mesencephalic reticular nuclei, wakefulness centers also involve histaminergic neurons in the posterior hypothalamus (tuberomammillary nucleus or TMN), peptidergic cell groups (orexin/hypocretin and melanin-concentrating hormone or MCH) in lateral hypothalamic area (LHA)/perifornical area (PF), and cholinergic and GABAergic magnocellular neurons in the basal forebrain (nucleus basalis of Maynert in the substantia innominata, magnocellular preoptic nucleus, medial septal nucleus, nucleus of the diagonal band of Broca) (572).
Axons from the primary sleep center (VLPO) travel caudally towards the wakefulness centers of the posterior hypothalamus (TMN) and ponto-mesencephalic reticular formation (LC, DR) via the medial forebrain bundle (MFB) (582). Their cells of origin can be traced primarily to the VLPO core (VLPOc), but also extend to the periphery of this nuclear group (VLPOex) that innervate the LC, DR and mesopontine tegmentum (LDT and PPT). Synaptic contacts in the LC and DR are primarily established by galanin-containing and to a lesser extent, GABAergic VPLO inputs (583-586). VPLO synaptic contacts in the LDT and PPT are made with interneurons, likely disinhibiting inhibitory influences originating in TMN, LC and DR. The signals are then directed to the principal cholinergic cells of the mesopontine tegmentum (557,558).
Fibers from the secondary sleep center (thalamic DM and reticular nuclei) course either in the periventricular system or in the MFB (thalamic peduncle of the ansa peduncolaris) to reach the periventricular and lateral preoptic hypothalamus (582) and mesopontine tegmental nuclei (588), respectively. Some of these axons contain glutamate and may enter the corona radiata to innervate the prefrontal cortex, that reproject back to the lateral hypothalamus (588).
Axons from the wakefulness cell groups (ponto-mesencephalic, hypothalamic and telencephalic) enter the MFB and travel rostrally through 2 pathways. The first is through a dorsal route, mainly provided by cholinergic mesopontine tegmental nuclei (LDT and PPT) and, to a lesser extent, by cholinergic and GABAergic basal forebrain neurons. Axons innervate primarily the intralaminar and reticular thalamic nuclei, that diffusely reproject to the cortex. The second is through a ventral route arising from aminergic/glutamatergic pontine and mesencephalic nuclei (LC, PC, DR-PBN, vPAG), aminergic (histamine) and peptidergic (orexin/hypocretin and MCH) cell groups in the posterior and lateral hypothalamus (TMN and LHA/PF, respectively), as well as by the majority of cholinergic and GABAergic basal forebrain neurons. These fibers cross the lateral hypothalamus and basal forebrain to reach all cortical areas (576,589). However, only the PBN and paracoeruleus (PC) glutamateric projections diffusely innervate the magnocellular nuclei in the basal forebrain (590). Finally, peptidergic (orexin/hypocretin and MCH) neurons in the LHA/PF also enter the MFB to heavily innervate aminergic and cholinergic cell groups in the posterior hypothalamus (TMN), pons-mesencephalon (LC, DR) and pontine tegmentum (LDT, PPT), respectively (564).
Initiation of sleep and maintenance of deep sleep are driven by the VLPO, via inhibition of monoaminergic centers in the posterior hypothalamus (TMN) and ponto-mesencephalic reticular formation (primarily LC and DR). Rodents with excitotoxic lesions of the VLPO show a reduction of NREM sleep that closely correlates with the loss of Fos-immunoreactive neurons in the VLPOc (586,587). Indeed, pontine and mesencephalic reticular nuclei (including the vPAG) and the cholinergic basal forebrain cells are responsible for electrical brain resynchronization, arousal and the waking state, through their excitatory dorsal- and ventral-projecting axonal pathways to the cortex (591,592). Specifically, glutamatergic projections from the PBN and PC, mediated by basal forebrain centers, play a key role in maintaining wakefulnees and REM sleep depending on activation or inhibition of the ascending monoaminergic arousal system (590). Such a possibility is consistent with clinical evidence that in humans, long lasting coma and a persistent vegetative state correlate with hemorrhage in the PBN region (593). In contrast, reactivation of the PBN-PC complex by lesions of the inhibitory, mesopontine tegmentum, may trigger dream-like states with intense visual experiences in awake subjects at inappropriate times (so called peduncolar hallucinosis), mimicking cortical REM-like behavior in a state of vigilance (594).
Orexin/hypocretin cells in the LHA/PF contribute to the waking state by stimulating the posterior hypothalamic (TMN) and ponto-mesencephalic (LC, DR) aminergic waking centers, thus reinforcing cortical arousal. Excitatory orexin 1 and 2 receptors have been found in all brainstem and basal forebrain wakefulness centers (595,596). Injection of orexin/hypocretin into these areas increases neuronal firing (597,598), while its administration into the preoptic hypothalamus presynaptically inhibits VLPO excitability (599). In addition, gene knockout for orexin/hypocretin, mutations in the orexin 2 receptor gene or absence of CSF orexin are associated with narcolepsy in mammals and man (600-602).
The VLPO also stimulates cholinergic neurons in the LDT and PPT to induce REM bursts, thus favoring arousal without wakefulness. In particular, outputs from LDT and PPT (and possibly from part of the basal forebrain cells) are excitatory to thalamic neurons projecting to the cortex. When the VLPO activates the reticular pontotegmental nuclei, the transthalamic sensory transmission may easily diffuse to the cortical mantle, leading to enhancement of cortical arousal during conditions of synchronized brain activity (NREM to REM shift) (576,591). Conversely, loss of Fos-immunoreactive neurons in the VLPOex correlates with a reduction in REM sleep episodes (594,595).
In contrast, cholinergic neurons in the basal forebrain (medial septum and diagonal band of Broca) are implicated in the generation of specific electrical activity during REM episodes (theta waves) in response to stimulation by brainstem aminergic inputs (603) such as from the PBN-PC complex (590). Also, MCH neurons in the LHA/PF contribute to the REM sleep by inhibiting the ponto-mesencephalic monoaminergic inputs to the cortex. This inhibition amplifies arousal without wakefulness (REM phase) mediated by mesopontine tegmental centers (LDT and PPT) (576). A reciprocal, negative feedback circuitry is established between pontine aminergic cell groups (LC area and tegmental area), leading to episodic switch between NREM and REM phases (605). Finally, thalamic DM and reticular neurons likely come into play to coordinate either REM or NREM sleep with other behavioral and endocrine regulations (605), as well as to increase arousal threshold by reinforcing the inhibitory action of the VLPO on rostral mesencephalic waking centers (580). Consistently, excitotoxic lesions of the thalamic DM induce persistent insomnia in cats (606) and its degeneration in humans gives rise to fatal familial insomnia, a disorder characterized by loss of rhythmicity in sleep-wake cycle, body temperature, blood pressure and anterior pituitary secretions (607). Figure 41 shows a simplified and integrated view of the neural circuitry controlling the mammalian sleep-wake cycle.
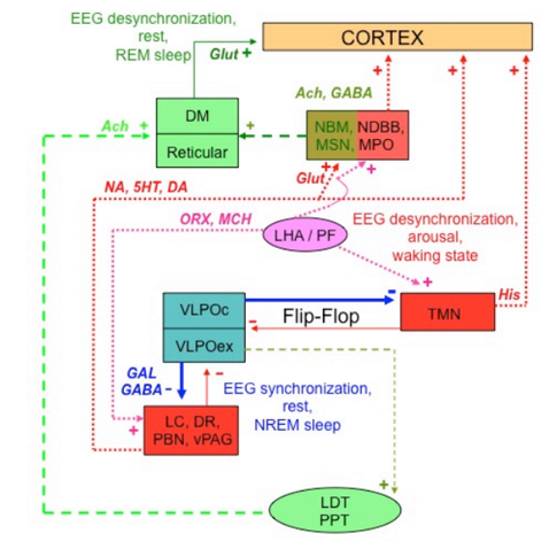
Figure 41. Schematic drawing showing a simplified and integrated view of the neural circuitry controlling the mammalian sleep-wake cycle. Different colors highlight the pathways for wakefulness (red and pink), NREM sleep (blue) and REM sleep (green). Continuous lines indicate primary circuitries for initiation of the sleep and waking state, dotted and hatched lines permissive circuitries for different sleep phases (NREM vs REM) and behavioral arousal, mixed colors different patterns of activity of the same center in relation to either sleep phases or wakefulness. Ach = acetylcholine; DA = dopamine; NDBB = nucleus of the diagonal band of Broca; DM = thalamic dorsomedial nucleus; DR = dorsal raphe; GABA = aminobutyric acid; GAL = galanin; Glut = glutamic acid; His = histamine; 5HT = 5-hydroxytryptamine or serotonin; LC = locus coeruleus; LDT = laterodorsal tegmental nucleus; MCH = melanocortin-concentrating hormone; MPO = magnocellular preoptic nucleus; MSN = medial septal nucleus; NA = noradrenaline; NBM = nucleus basalis of Maynert; ORX = orexin/hypocretin; PBN = parabrachial nucleus; PPT = peduncolopontine tegmental nucleus; Reticular = thalamic reticular nucleus; vPGA = ventral periacqueductal grey; VPLO = hypothalamic ventrolateral preoptic nucleus, VPLOc = VPLO core; VPLOex = VPLO extended; TMN = hypothalamic tuberomammillary nucleus; + = stimulation; - = inhibition
Major regulators of the neural machinery for the sleep-wake cycle are the circadian timing system (CTS), feedback regulation exerted by locomotor activity and sleep-wake cycle itself onto the CTS, and homeostatic mechanisms endogenous to the sleep and wakefulness neural circuitries (sleep homeostat) (576) including the activity of the glymphatic system (212). The CTS is primarily governed by the hypothalamic suprachiasmatic nucleus (SCN) and its widespread CNS projections, including those that trigger melatonin secretion from the pineal gland (see section F. Circadian Rhythmicity). In rats, sleep-wake rhythmicity is adaptively coordinated with feeding behavior. In fact, light sensitive, SCN projections to the ventral subparaventricular zone (608) innervate food-entrainable neurons in the hypothalamic dorsomedial nucleus (608,609) that sends stimulatory glutamate-containing inputs to LHA orexin neurons and inhibitory GABAergic inputs to VLPO neurons (610). In this manner, photic-dependent stimuli can be integrated with nonphotic stimuli to establish a rest/sleep-locomotion/wakefulness cycle that is ideal for nutritional success (611). In turn, locomotor activity may feed back onto the SCN by activating NPY-containing outputs in the intergeniculate leaflet of the thalamus and serotoninergic projections in the median raphe nucleus to entrain the sleep-wake cycle with levels of exploratory behavior (612). In humans, disruption of this locomotor-circadian rhythm circuitry has been implicated as a potential cause for development of cognitive decline and neurodegeneration. In support, recent evidence in Drosophila shows that stable, long-term memory depends on circadian cycles in rest and activity, leading to impairment in memory consolidation when sleep disruption occurs. In fact, increased locomotion and waking state results in loss of synaptic remodeling at critical brain sites (613) such as the hypocretin/orexin system in the LHA where the number of synapses oscillates with light and dark phases (614). In addition, stress has been associated with increased cFos expression in a number of limbic areas (infralimbic cortex, central nucleus of the amygdala, bed nucleus of the stria terminalis) projecting to both activating (VLPO) and inhibiting (LC, TMN) sleep centers. These inputs may be important for maintaining a waking state during behavioral arousal, such as an emergency occurring during normal sleep. Their activation by anxiety may contribute to stress-induced insomnia (615). Hyperactivation of corticolimbic sites has also been shown in human subjects with insomnia and might contribute to the excessive high frequency EEG activity seen during NREM sleep, including their sensation of being awake even when the EEG appears to be in NREM, a condition known as “sleep state misperception” (616).
Finally, some cellular and molecular mechanisms may serve as an internal homeostat to inhibit aminergic waking centers and activate the VLPO (613,617). In particular, in conditions of sleep deprivation, rat astrocytes have been found to release adenosine in the perineuronal space of LC and orexigenic LHA/PF to shut off monoaminergic excitatory systems via A1 inhibitory adenosine receptors. This inhibition of noradrenergic arousal mechanisms would also favor activation of the hypothalamic glymphatic flow by increasing the interstial space, and relieving the restraining effect of catecholaminergic inputs to the choroid plexus, resulting in increased CSF production. Thus, neurotoxic waste products could be more removed, and the brain neurons cleared as during a regular sleep (212). Extraneuronal adenosine may also reduce presynaptic inhibition of VLPO neurons via A2 excitatory adenosine receptors, thereby triggering compensatory sleep (613).
This and a number of circulating endocrine, metabolic and immune inputs controlled by the CTS may influence circadian clock genes (576,618) that in turn, influence the sleep-wake cycle. In particular, knockout mice for Clock, Cry1/Cry2, and Bmal1 in the SCN and related neural pathways show either an increase (Clock) or decrease (Cry and Bmal1) in NREM sleep (619-621). In rodents, Clock and Bmal1 deactivation also reduces orexin outputs in the LHA, giving rise to a hyperphagic, obese and dysmetabolic phenotype. Other gene candidates involved in this regulation include REV-Erba1, whose impairment leads to altered locomotor activity in either constant light or darkness, and PGC-1α / PGC-1β, that control metabolic rate in liver and muscle, body temperature and locomotor activity in the dark phase (622). Collectively, clock gene activation / deactivation phases by the CTS during the sleep-wake cycle may play a key role in regulating synaptic plasticity and metabolic balance.
REFERENCES
- Christ JF. 1969 Derivation and boundaries of the hypothalamus, with atlas of hypothalamic grisea, in Haymaker W, Anderson E, Nauta WJH (eds): The Hypothalamus. Charles C. Thomas, pp 13-60.
- May MT. 1968 Galen: On the usefulness of the parts of the body.De Usu Partium. Cornell University Press, pp 424- 461.
- Toni R.2000 Ancient views on the hypothalamic-pituitary-thyroid axis: an historical and epistemological perspective. Pituitary 3: 83-95.
- Meshberger FL.1990 An interpretation of the Michelangelo’s Creation of Adam based on neuroanatomy. JAMA 10: 1837-1841.
- His W.1893 Vorschlage zur Einteilung des Gehirns. Arch Anat Physiol, Anat Alb 172-179.
- Toni R, Malaguti A, Benfenati F, Martini L. The human hypothalamus: a morphofunctional perspective. J Endocrinol Invest 27 (supp to n.6), 73-94, 2004.
- Ciocca DR, Puy LA, Stati AO. 1984 Constitution and behavior of follicular structures in the human anterior pituitary gland. Am J Pathol 115:165-174.
8.Horvath E, Kovacs K. 2002 Folliculo stellate cells of the human pituitary: a type of adult stem cells? Ultrastruct Pathol 26: 219-228.
- Fitzgerald KT. 1979 The structure and function of the pars tuberalis of the vertebrate adenohypophysis. Gen Comp Endocrinol 37: 383-399.
- Cohen LE, Radovick S. 2002 Molecular basis of combined pituitary hormone deficiencies. Endocr Rev 23: 431-442.
- Zhu X, Gleiberman AS, Rosenfeld MG.2007 Molecular physiology of pituitary development: signaling and transcriptional networks. Physiol Rev 87: 933-963.
- Zhu X, Wang J, Ju B-G, Rosenfeld MG. 2007 Signalling and epigenetic regulation of pituitary development. Curr Op Cell Biol 19: 605-611.
- Alatzoglou KS, Kelberman D, Dattani MT.2009 The role of SOX proteins in normal pituitary development.J. Endocrinol 200, 245–258.
- Nakane PK. 1970 Classifications of anterior pituitary cell types with immunoenzyme histochemistry. J Histochem Cytochem 18: 9-20.
- Snyder G, Hymer WC, Snyder J. 1977 Functional heterogeneity in somatotrophs isolated from the rat anterior pituitary. Endocrinology101: 788-799.
- Nikitovitch-Winer MB, Atkin J, Maley BE. 1987 Colocalization of prolactin and growth hormone within specific adenohypophyseal cells in male, female and lactating female rats. Endocrinology121: 625-630.
- Frawley LS, Clark CL, Schoderbek WE, Hoeffler JP, Boockfor FR. 1986 A novel bioassay for lactogenic activity: Demonstration that prolactin cells differ from one another in bio-and immuno-potentencies of secreted hormone. Endocrinology119: 2867-2869.
- Schwartz J, Canny B, Vale WW, Funder JW. 1989 Intrapituitary cell-cell communication regulates ACTH secretion, Neuroendocrinology 50: 716-722.
- Nakajima T, Yamaguchi H, Takahashi K. 1980 S100 protein in folliculostellate cells of the rat pituitary anterior lobe. Brain Res191: 523-531.
- Denef C. 1994 Paracrine mechanisms in the pituitary, in Imura H (ed): The Pituitary Gland. Raven Press, pp 351-378.
- Vankelecom H.2007 Non-hormonal cell types in the pituitary candidating for stem cell. Sem in Cell Develop Biol 18: 559-570.
- Rizzoti K. 2010. Adult pituitary progenitors/stem cells: from in vitro characterization to in vivo function.Eu. J. Neurosci. 32: 2053–2062.
- Garcia-Lavandeira M, Quereda V, Flores I, Saez C, Diaz-Rodriguez E, Japon MA, Ryan AK, Blasco MA, Dieguez C, Malumbres M, Alvarez CV 2009 A GRFa2/Prop1/stem (GPS) cell niche in the pituitary. PloS One 4:e4815.
- Bergland RM, Page RB. 1978 Can the pituitary secrete directly to the brain? Endocrinology102:1325-1338.
- Leclercq T, Grisoli F. 1983 Arterial blood supply of the normal human pituitary gland. J Neurosurg 58: 678-681.
- Porter JC, Kamberi IA, Grazia YR. 1978 Pituitary blood flow and portal vesels, in Martini L, Ganong W (eds): Frontiers in Neuroendocrinology. Oxford University Press, pp 145-175.
- Gross PM, Joneja M, Pang JJ, Polischuk TM, Shavers SW, Wainman DS. 1993 Topography of short portal vessels in the rat pituitary gland: a scanning electron-microscopic and morphometric study of corrosion cast replicas. Cell Tiss Res 242: 79-88.
- Yuh WTC, Fisher DJ, Nguyen HD, Tali ET, Gao F, Simonson TM, Schlechte JA. 1994 Sequential MR enhancement pattern in normal pituitary gland and in pituitary adenoma. AJNR 15: 101-108.
- Wittkowski WH, Schulze-Bonhage AH, Bockers TM. 1992 The pars tuberalis of the hypophysis: A modulator of the pars distalis? Acta Endocrinologica126: 285-290.
- Iliff JJ, Lee H, Yu M, Feng T, Logan J, Nedergaard M, Benveniste H. 2013 Brain-wide pathway for waste clearance captured by contrast-enhanced MRI. J Clin Invest. 123:1299-309.
- Altman J, Bayer SA. 1986 Development of the rat hypothalamus. Adv. Anat. Embriol. Cell Biol 85.
- Markakis E. A. 2002 Development of the neuroendocrine hypothalamus. Frontiers in Neuroendocrinoloty 23: 257-291.
- Christ J. F. 1969 Derivation and boudaries of the hypothalamus, with atlas of hypothalamic grisea, in Haymaker W, Anderson E, Nauta WJH (eds): The Hypothalamus. Charles C. Thomas, pp 13-60.
- Wataya T, Ando S, Maguruma K, Ikeda H, Watanabe K, Eiraku M, Kawada M, Takahashi J, Hashimoto N, Sasai Y.2008 Minimization of exogenous signals in ES culture induces rostral hypothalamic differentiation. Proc Natl Acad Sci 105: 11796-11801.
- Tessmar-Raible K, Raible F, Christodoutou F, Guy Keren, Rembold M, Hausen H, Arendt D.2007 Conserved sensory-neurosecretory cell types in annelid and fish forebrain: Insights into hypothalamic evolution. Cell 129: 1389-1400.
- Sauer F. C. 1935 Mitoses in the neural tube. J. Comp. Neurol. 62: 377-405.
- Ohyama K, Das R, Placzek M.2008 Temporal progression of hypothalamic patterning by a dual action of BMP Development 135, 3325-3331.
- Figdor MC, Stern CD. 1993 Segmetnal organization of the embryonic diencephalon. Nature 363: 630-634.
- Coggeshall RE. 1964 A study of diencephalic development in the albino rat. J. Comp. Neurol. 122: 241-270.
- Koutcherov Y, Mai JK, Ashwell KWS, Paxinos G.2002 Organization of human hypothalamus in fetal development. J Comp Neurol 446: 301-324.
- Markakis EA, Swanson L.W. 1997 Spatiotemporal patterns of secretomotor neuron generation in the parvicellular neuroendocrine system.Brain Res. Rev. 24: 255-291.
- Schwanzel-Fukuda M, Pfaff DW. 1989 Origin of luteinizing hormone-releasing hormone neurons. Nature 338: 161-164.
- Toni R, Briganti F., Lechan RM, Vezzadini P. 1996 Immunocytochemical distribution of somatostatin in the hypothalamus of the human fetus. Abstracts 10th International Congress of Endocrinology, S. Francisco, p.292.
- Toni R, Musolino A, Ruggeri F, Scialpi C. 1996 Immunocytochemical distribution of thyrotropin-releasing hormone (TRH) prohormone in the diencephalon of the human fetus. It. J. Anat. Embriol. 101 (supp):2.
- Alvarez-Bolado G, Rosenfeld MG, Swanson LW. 1995 Models of forebrain regionalization based on spatiotemporal patterns of POU-III homeobox gene expression, birthdates and morphological features. J. Comp. Neurol. 355: 237-295.
- Nakai S, Kawano H, Yudate T, Nishi M, Kuno J, Nagata A, Jishage K, Hamada H, Fujii H, Kawamura K, Shiba K, Noda T. 1995 The POU domain transcription factor Brn-2 is required for the determination of thedevelopmen of the rat somatostatin-containing neuocrotical neuronal system. Genes Dev. 9: 3109-3121.
- Schonemann MD, Ryan A K, McEvilly RJ, O’Connell SM, Arias CA, Kalla KA, Li P, Sawchenko PE, Rosenfeld MG. 1995 Development and survival of the endocrine hypothalamus and posterior pituitary glnd requires the neuronal POU domain factro Brn-“. Genes Dev. 9: 3122-3135.
- Michaud J.L., Rosenquist T, May NR, Fan C-M. 1998 Development of neuroendocrine lineages require the bHLH-PAS transcription factor SIM1. Genes Dev. 12: 3264-3275.
- Norsted E, Gomuc B, Meister B. 2008 Protein components of the blood-brain barrier (BBB) in the mediobasal hypothalamus, J Chem Neuroanat. 36: 107-121.
- Michaud JL, DeRossi C, May NR, Holdener BC, Fan C-M. 2000 ARNT2 acts as the dimerization partner of SIM1 for the development of the hypothalamus. Mech Dev. 90: 253-261.
- lle Duplan SM, Bouche F, Alexandrov L, Michaud JL. 2009 Impact of Sim1 gene dosage on the development of the paraventricular and supraoptic nuclei of the hypothalamus.Eu. J. Neurosci. 2239–2249, 2009.
- Tobet ST.2002 Genes controlling hypothalamic development and sexual differentiation. Eu J Neurosci 16: 373-376.
- Kurrasch DM, Cheung CC, Lee FY, Tran PV, Hata K, Ingraham HA.2007 The neonatal ventromedial hypothalamus transcriptome reveals novel markers with spatially distinct pattering. J Neurosci 27: 13624-13634.
- Ivanova E, Kelsey G. 2011 Imprinted genes and hypothalamic function.J Mol Endocrinol 47; R67-R74.
- Swanson L.W. 1987 The hypothalamus, in Bjorklund A. Hokfelt T, Swanson LW (eds), Handbook of Chemical Neuroanatomy, vol 5, Integrated Systems of the CNS, part 1, Elsevier Science Publisher, pp 1-124.
- Thomson RH, Swanson LW. 2003 Structral characterization of a hypothalamic visceromotor pattern generator network.Brain Res Rev 41: 153-202.
- Weindl A. 1973 Neuroendocrine aspects of circumventricular organs, in Ganong W, Martini L (eds): Frontiers in Neuroendocrinology, Oxford University Press, pp 3-32.
- Oldfield BJ, McKinley MJ. 1995 Circumventricular organs in Paxinos G (ed) The Rat Nervous System, Academic Press, pp 391-403.
- Johnson AK, Gross PM. 1993 Sensory circumventricular organs and brain homeostatic pathways. FASEB J 7: 678-686.
- Monroe BG. 1967 A comparative study of the ultrastructure of the median eminence, infundibular stem and neural lobe of the hypophysis of the rat. Zeitschrift fur Zellforschung 76: 405-432.
- Knigge KM, Scott DE. 1970 Structure and function of the median eminence. Am J Anat 129: 223-244.
- Ugrumov MV. 1992 Development of the median eminence during ontogenesis (morpho-functional aspects). in Ermisch A, Landgraf R, Ruhle H-J (eds): Progress in Brain Research, vol 91, pp 349-356.
- Petrov T, Howarth AG, Krukoff TL, Stevenson BR. 1994 Distribution of the tight junction-associated protein ZO-1 in circumventricular organs of the CNS. Mol Brain Res 21: 235-246.
- Krisch B, Leonhardt H. 1978 The functional and structural border of the neurohemal region of the median eminence. Cell Tissue Res 192: 327-339.
- Rodriguez EM, Blazquez JL, Pastor FE, Pelaez B, Peruzzo B, Amat P. 2005. Hypothalamic tanycytes: a key component of brain-endocrine interaction. Int Rev cytol 247: 89-164.
- Lechan RM, Fekete C. 2007 Infundibular tanycytes as modulators of neuroendocrine function: hypothetical role in the regulation of the thyroid and gonadal axis. Acta Biomed 78: 84-98.
- Wittmann G, Farkas E, Szilvasy-Szabo A, Gereben B, Fekete C, Lechan RM. 2016. Variable proopiomelanocortin expression in tanycytes of the adult rat hypothalamus and pituitary stalk. J Comp Neurol, in press.
- Scott DE, Paull WK. 1979 The tanycyte of the rat median eminence. Cell Tissue Res 200: 329-334.
- King J, Rubin BS. 1994 Dynamic changes in LHRH neurovascular terminals with various endocrine conditions in adults. Hormones and Behavior 28:349-356.
- Yoshimura T, Yasuo S, Watanabe M, Iigo M, Yamamura T, Hirunagi K, Ebihara S. 2003 Light-induced hormone conversion of T4 to T3 regulates photoperiodic response iof gonads in birds. Nature 13: 178-181.
- Beagley GH, Hatton GI. 1992 Rapid morphological changes in supraoptic nucleus and posterior pituitary induced by a single hypertonic saline injection. Brain Res Bull 28: 613-618.
- Ondo JG, Mical RS, Porter JC. 1972 Passage of radioactive substances from CSF to hypophysial portal blood. Endocrinology 91: 1239-1246.
- Kendall JW, Jacobs JJ, Kramer RM. 1972 Studies on the transport of hormones from the cerebrospinal fluid to hypothalamus and pituitary. in Knigge KM, Scott DE, Weindl A (eds) Brain-Endocrine Interaction. Median Eminence: Structure and Function, Karger, Basel, pp. 342-349.
- Kozlowski GP, Coates PW. 1985 Ependymoneural specializations between LHRH fibers and cells of the cerebroventricular system. Cell Tiss Res 242: 301-311.
- Tu HM, Kim S-W, Salvatore D, Bartha T, Legradi G, Larsen PR, Lechan RM. 1997 Regional distribution of type 2 thyroxine deiodinase messenger ribonucleic acid in rat hypothalamus and pituitary and its regulation by thyroid hormone. Endocrinology 138: 3359-3368.
- Fekete C, Freitas BC, Zeold A, Wittmann G, Kadar A, Liposits Z, Christoffolete MA, Singru P, Lechan RM, Bianco AC, Gereben B. 2007 Expression patterns of WSB-1 and USP-33 underlie cell-specific posttranslational control of type 2 deiodinase in the rat brain. Endocrinology 148: 4865-4874.
- Kallo I, Mohacsik P, Vida B, Zeold A, Bardoczi Z, Zavacki AM, Farkas E, Kadar A, Hrabovszky E, Arrojo E, Drigo R, Dong L, Barna L, Palkovitz M, Borsay BA, Herczeg L, Lechan RM, Bianco AC, Liposits Z, Fekete C, Gereen B. 2012 A novel pathway regulates thyroid hormone availability in rat and human hypothalamic neurosecretory neurons. PLoS One 7: e37860.
- Sanchez, E., M. A. Vargas, P. S. Singru, I. Pascual, F. Romero, C. Fekete, J. L. Charli and R. M. Lechan (2009). "Tanycyte pyroglutamyl peptidase II contributes to regulation of the hypothalamic-pituitary-thyroid axis through glial-axonal associations in the median eminence." Endocrinology 150(5): 2283-2291.
- Freitas, B. C., B. Gereben, M. Castillo, I. Kallo, A. Zeold, P. Egri, Z. Liposits, A. M. Zavacki, R. M. Maciel, S. Jo, P. Singru, E. Sanchez, R. M. Lechan and A. C. Bianco (2010). "Paracrine signaling by glial cell-derived triiodothyronine activates neuronal gene expression in the rodent brain and human cells." J Clin Invest 120(6): 2206-2217;
- Ebling, F. J. P. and J. E. Lewis (2018). "Tanycytes and hypothalamic control of energy metabolism." Glia 66(6): 1176-1184
- Farkas, E., E. Varga, B. Kovacs, A. Szilvasy-Szabo, A. Cote-Velez, Z. Peterfi, M. Matziari, M. Toth, D. Zelena, Z. Mezricky, A. Kadar, D. Kovari, M. Watanabe, M. Kano, K. Mackie, B. Rozsa, Y. Ruska, B. Toth, Z. Mate, F. Erdelvi, G. Szabo, B. Gereben, R. M. Lechan, J. L. Charli, P. Joseph-Bravo and C. Fekete (2020). "A glial-neuronal circuit in the median eminence regulates thyrotropin-releasing hormone-release via the endocannabinoid system." iScience 23(3): 100921.
- Lee DA, Bedoni JL, Pak T, Wang H, Song J, Miranda-Anquio A, Takiar V, Charubhumi V, Balordi F, Takebayashi H, Aja S, Ford E, Fishell G, Blackshaw S. 2012. Tanycytes of the hypothalamic median eminence form a diet-responsive neurogenic niche. Nat Neurosci 15: 700-702.
- Sanders NM, Dunn-Meynell AA, Levin BE. 2004. Third ventricular alloxan reversible impairs glucose counterregulatory responses.Diabetes 53: 1230-1236.
- Wittmann G, Farkas E, Szilvasy-Szabo A, Gereben, Fekete C, Lechan RM, Variable proopiomelanocortin expression in tanycytes of the adult rat hypothalamus and pituitary stalk. J Comp Neurol, in press.
- Robins SC, Stewart I, McNay DE, Taylor V, Giachino C, Goetz M, Ninkovic J, Briancon N, Maratos-Flier E, Flier JS, Kokoeva MV, Placzek M. 2013. A-Tanycytes of the adult hypothalamic third ventricle include distinct populations of FGF-responsive neural progenitors. Nat Commun 4: 1-13.
- Clarke I, Jessop D, Millar R, Morris M, Bloom S, Lightman S, Cohen CW, Lew R, Smith I. 1993 Many peptides that are present in the external zone of the median eminence are not secreted into the hypophysial portal blood of sheep. Neuroendocrinology 57:765-775.
- Merchenthaler I, Gorcs T, Setalo G, Petrusz P, Flerko B. 1984 Gonadotropin-releasing hormone (GnRH) neurons and pathways in the rat brain. Cell Tissue Res 237: 15-29.
- Kuljis RO, Davis JP. 1989 Immunocytochemical and physiological evidence of synapse between dopamine- and luteinizing-hormone releasing hormone-containing neurons in the ewe median eminence. Endocrinology124: 1579-1581.
- Contijoch AM, Gonzalez C, Singh HN, Malamed S, Troncoso S, Advis J-P. 1992 Dopaminergic regulation of luteinizing hormone-releasing hormone release at the median eminence level: Immunocytochemical and physiological evidence in hens. Neuroendocrinology 55:290-300.
- Sahu A, Crowley WR, Tatemoto K, Balasubramaniam A, Kalra SP. 1987 Effects of neuropeptide Y, NPY analog (norleucine4-NOY), galanin and neuropeptide K on LH release in ovariectomized (OVX) and OVX estrogens, progesterone-treated rats. Peptides 8:921-926.
- Lopez FJ, Negro-Vilar A. 1990 Galanin stimulates luteinizing hormone-releasing hormone secretion from arcuate nucleus-median eminence fragments in vitro: Involvement of an alpha-adrenergic mechanism. Endocrinology 127:2431-2436.
- Gross PM. 1992 Circumventricular organ capillaries. Prog Brain Res 1:219-233.
- Sutton SW, Toyama TT, Otto S, Plotsky PM. 1988 Evidence that neuropeptide Y (NPY) released into the hypophysial-portal circulation participates in priming gonadotropes to the effects of gonadotropin releasing hormone (GnRH). Endocrinology 123:1208-1210.
- Sahu A, Crowley WR, Kalra SP. 1994 Hypothalamic neuropeptide-Y gene expression increases before the onset of the ovarian steroid-induced luteinizing hormone surge. Endocrinology 134:1018-1022.
- Mezey E, Kiss JZ. 1985 Vasoactive intestinal polypeptide containing neurons in the paraventricular nucleus may participate in regulating prolactin secretion. Proc Natl Acad Sci USA 82:245-247.
- Stillman MA, Recht LD, Rosario SL, Seif SM, Robinson AG, Zimmerman EA. 1977 The effects sof adrenalectomy and glucocorticoid replacement on vasopressin and vasopressin-neurophysin in the zona externa of the median eminence of the rat. Endocrinology 101: 42-49.
- King J, Letourneau RJ. 1994 Luteinizing hormone-releasing hormone terminals in the median eminence of rats undergo dramatic changes after gonadectomy, as revealed by electron microscopic image analysis. Endocrinology 1994; 134: 1340-1351.
- Villar MJ, Meister B, Hokfelt T. 1994 Reorganization of neural peptidergic systems in the median eminence after hypophysectomy. J Neurosci 14:5996-6012.
- Liao N, Bulant M, Nicolas P, Vaudry H, Pelletier G. 1988 Electron microscope immunocytochemical localization of thyrotropin-releasing hormone (TRH) prohormone in the rat hypothalamus. Neuropeptides 11:107-110.
- Bulant M, Roussel J-P, Astier H, Nicolas P, Vaudry H. 1990 Processing of thyrotropin-releasing hormone prohormone (pro-TRH) generates a biologically active pepide, prepro-TRH-(160-169), whch regulates TRH-induced thyrotropin secretion. Proc Natl Acad Sci USA 87:4439-4443.
- Niimi M, Takahara J, Sato M, Kawanishi K. 1990 Immunohistochemical identification of galanin and growth hormone-releasing factor-containing neurons projecting to the median eminence of the rat. Neuroendocrinology 51:572-575.
- Ottlecz A, Samson WK, McCann SM. 1986 Galanin: Evidence for a hypothalamic site of action to release growth hormone. Peptides 7:51-53
- Davis TME, Burrin JM, Bloom SR. 1987 Growth hormone (GH) release in response to GH-releasing hormone in man is 3-fold enhanced by galanin. J Clin Endocrinol Metab 65:1248-1252.
- Lechan RM, Wu P, Jackson IMD, Wolfe H, Cooperman S, Mandel G, Goodman RH. 1986 Thyrotropin-releasing hormone precursor: Characterization in rat brain. Science 231:159-161.
- Ceccatelli S, Eriksson M, Hokfelt T. 1989 Distribution and coexistence of corticotropin-releasing factor-, neurotensin-, enkephalin-, cholecystokinin-, galanin- and isoleucine-like peptides in the parvocellular part of the paraventricular nucleus. Neuroendocrinology 9:309-323.
- Hokfelt T, Fahrenkrug J, Ju G, Ceccatelli S, Tsuruo Y, Meister B, Mutt V, Rundgren M, Brodin E, Terenius L, Hulting A-L, Werner S, Bjorklund H, Vale W. 1987 Analysis of peptide histidine-isoleucine and vasoactive intestinal polypeptide-immunoreactive neurons in the central nervous system with special reference to their relation to CRF- and enkephalin-like immunoreactivities in the paraventricular hypothalamic nucleus. Neuroscience 23: 827-857.
- Ceccatelli S, Lundberg JM, Fahrenkrug J, Bredt DS, Snyder SH, Hokfelt T. 1992 Evidence for involvement of nitric oxide in the regulation of hypothalamic portal blood flow. Neuroscience 51:769-772.
- Ceccatelli S, Fahrenkrug J, Villar MJ, Hokfelt T. 1991 Vasoactive intestinal polypeptide/peptide histidine isoleucine immunoreactive neuron systems in the basal hypothalamus of the rat with special reference to the portal vasculature: An immunohistochemical and in situ hybridization study. Neuroscience 43:483-502.
- Said SI, Mutt V. 1970 Polypeptide with broad biological activity: Isolation from small intestine. Science 169:1217-1218.
- Palmer RMJ, Ferrige AG, Moncada S. 1987 Nitric oxide release accounts for the biological activity of endothelium-derived relaxing factor. Nature 327:524-526.
- Weindl A., Joynt R., Rochester N. 1972 Ultrastructure of the ventricular walls. Ach. Neurol (Chicago) 26: 420-426.
- Mitro A, Palkovits M. 1981 Morphology of the rat brain ventricles, ependyma and periventricular structures. Bibliotheca Anatomica, 21, S. Karger.
- Del Brio MA, Riera P, Gracia JM, Alvariz-Uria M. 1990 Ultrastructural study of the cellular components of the organum vasculosum of the lamina terminalis in the rabbit (Oryctolagus cuniculus). J. Submicrosc. Cytol. Pathol. 22: 303-309.
- Nakay Y, Kudo J, Hashimoto A. 1980 Specific cell membrane differentiation in the tanycytes and glial cells of the organum vasculosum of the lamina terminalis in dog. J. Electron Microsc. 29: 144-150.
- King J.C. Anthony ELP. 1984 LHRH neurons and their projections in humans and other mammals: Species comparisons. Peptides (Supp 1), 195-207.
- Yamaguchi K, Morimoto A, Murakami N. 1993 Organum vasculosum laminae terminalis (OVLT) in rabbit and rat: topographic studies. J. Comp. Neurol. 330: 352-362.
- Weindl A, Sofroniew MV. 1981 Relation of neuropeptides to mammalian circumventricular organs, in Martin JB, Reichlin S, Bick KL (eds): Neurosecretion and Brain Peptides, Raven Press, pp. 303-320.
- Palkovits M, Mezey E, Ambach G, Kivoviks P. 1978 Neural and vascular connections between the organum vasculosum laminae terminalis and preoptic nuclei. Brain-Endocrine Interaction IIII. Neural Hormones and Reproduction, D.E. Scott, G.P. Kozlowski, A. Weindl, Eds, Karger, Basal, pp 63-65.
- Ciura S, Bourque CW. 2006 Transient receptor potential vanilloid 1 is required for intrinsic osmoreception in organum vasculosum lamina terminalis neurons and for normal thirst responses to systemic hyperosmolality. J Neuroscience 26: 9069-9075.
- Oldfield BJ, Badoer E, Hards DK, McKinley MJ. 1994 Fos production in retrogradely labelled neurons of the lamina terminalis following intravenous infusion of either hyporetonic saline or angiotensin II. Neuroscience 60: 255-262.
- McKinley MJ, Burns P, Colvill LM, Wade JD, Weisinger RS, Tregear GW. 1997 Distribution of Fos immunoreactivity in the lamina terminalis and hypothalamus induced by centrally administered relaxin in consciuos rats. J. Neuroendocrinol. 9: 431-437.
- Farrell MJ, Bowala TK, Gavrilescu M, Phillips PA, McKinley MJ, McAllen RM, Denton DA, Egan GF. 2011 Cortical activation and lamina terminalis functional connectivity during thirst and drinking in humans. Am J Physiol Regul Integr Comp Physiol 301: R623–R631.
- Hoffman GE, Gibbs FP. 1982 Deafferentation spares a subchiasmatic LHRH projection to the median eminence. Neuroscience 8: 1979-1993.
- Ambach G, Palkovits M, Szentagothai J. 1976 Blood supply of the rat hypothalamus. IV. Retrochiasmatic area, median eminence, arcuate nucleus. Acta Morphol Acad Sci Hung 24: 93-119.
- Hoffman GE, Lee W-S, Wray S.Gonadotropin-releasing hormone (GnRH).In Nemeroff CB Ex., Neuroendocrinology, Boca Raton, FL, CRC Press, 1992, 185-217. 126. Jansen HT, Kirbi JD, Cooke PS, Arambepola N, Iwamoto GA. 2007 Impact of neonatal hypothyroidism on reproduction in the male hamster, Mesocricetus auratus. Physiol Behav 90: 771-781.
- Toni R, Della Casa C, Castorina S, Cocchi D, Celotti F. 2005 Effects of hypothyroidism and endocrine disruptor-dependent non-thyroidal illness syndrome on the GnRH-gonadotroph axis of the adult male rat. J Endocrinol Invest 28 (suppl to no 11): 20-27.
- Dellmann HD. 1979 The subfornincal organ. Int Rev Cytol 58: 333-421.
- Dellmann HD. 1985 Fine structural organizatiojn of the subfornical organ. A concise review. Brain Res Bull 15: 71-78.
- Dellmann HD, Simpson JB. 1976 Regional differences in the morphology of the rat subfornincal organ. Brain Res 116: 389-400.
- Gross PM. 1992 Circumventricular ogan capillaries. Prog. Brain Res. 91: 219-233.
- Swanon LW, Mogenson GJ. 1981 Neural mechanisms for functional coupling of autonomic, endocrine and somatomotor responses in adaptative behavior. Brain Res. Reviews 3: 2-34.
- Tanaka J, Nojima K, Yamamuro Y, Saito H, Nomura M. 1993 Responses of subfornical organ neurons projecting to the paraventricular nucleus to hemorrhage. Brain Res 608: 141-144.
- Krause EG, Melhorn SJ, Davis JF, Scott KA, Ma LY, deKloet AD, Benoit SC, Woods SC, Sakai RR. 2008 AT1 receptors in the subfornical organ mediate the drinking and hypothalamic-pituitary-adrenal response to systemic isoproterenol. Endocrinology
- Lind RW. The subfornical organ: neural connections, in: Gross P. (ed), Circumventricular Organs and Body Fluids, vol 1, CRC Press, pp. 27-42.
- Bourque CW, Oliet SHR, Richard D. 1994 Osmoreceptors, osmoreception and osmoregulation. Frontiers in Neuroendocrinology 15: 231-274.
- Ferguson AV. 1992 Neurophysiological analysis of mechanisms for the subfornical organ and area postrema involvement in autonomic control. Prog. Brain Res. 91: 413-421.
- Toni R, Mosca S, Ruggeri F, Valmori A, Orlandi G, Toni G, Lechan RM, Vezzadini P. 1995 Effect of hypothyroidism on vasoactive intestinal polypeptide-immunoreactive neurons in the forebrain-neurohypophysial nuclei of the rat brain. Brain Res. 682: 101-115.
- Sakai K, Agassandian K, Morimoto S, Sinnayah P, Cassell MD, Davisson RL, Sgimund CD. 2007 Local production of angiotensin II in the subfornical organ causes elevated drinking. J Clin. Invest 117: 1088-1095.
- Kai A, Ono K, Kawano H, Honda E, Nakanishi O. Inenaga K. 2006 Galanin inhibits neuronal activity in the subfornical organ in rat slice preparation. Neuroscience 143: 769-777.
- Samson WK, White MM, Price C, Ferguson AV.2007 Obestatin acts in brain to inhibit thirst. Am J Physiol Regul Integr Comp Physiol 292: R637-R643.
- Hajdu I, Szentirmai E, Obai Jr F, Krueger JM.2003 Different brain structures mediate drinking and sleep suppression elicited by the somatostatin analog octreotide in rats. Brain Res 994: 115-123.
- Toni R, Mosca S, Lechan RM, Jackson IMD, Vezzadini P. 1994 Immunocytochemical distribution of the thyrotropin-releasing hormone (TRH) prohormone (ProTRH) and its terminal peptides in the rat subfornical organ. Eu J Endocrinoll 130 (suppl 2): P4.073, 251.
- Young CN, Morgan DA, Butler SD, Mark AL, Davisson RL. 2013 The brain subfornical organ mediates leptin-induced increases in renal sympathetic activity but not its metabolic effects. Hypertension. 6:737-44.
- Gross PM. 1985 The subfornical organ as a model of neurohumoral integration. Brain Res. Bull. 15: 65-70
- Nauta SJH, Haymaker W. 1969 Hypothalamic nuclei and fiber connections, in Haymaker W, Anderson E, Nauta WJH (eds): The Hypothalamus. Charles C. Thomas, pp. 136-209
- Swanson LW. 1987 The hypothalamus, in Bjorklund A. Hokfelt T, Swanson LW (eds), Handbook of Chemical Neuroanatomy, vol 5, Integrated Systems of the CNS, part 1, Elsevier Science Publisher, pp 1-124.
- Vergani F, Landi A, Antonini A, Parolin M, Cilia R, Grimaldi M, Ferrarese C, Gaini SM, Sganzerla EP. 2007 Anatomical identification of active contacts in subthalamic deep brain stimulation.Mov Disord 18: 1337-1340.
- Novakova L, Ruzika E, Jech R, Serranova T, Dusek P, Urgosik D.2007 Increase in body weight in a non-motor side effect of deep brain stimulation of subthalamic nucleus in Parkinson’s disease. Neuro Endocrinol Lett 28: 21-25.
- Szentagothai J. 1964 The parvicellular neurosecretory system, in Bargmann W, Shale JP (eds), Progress in Brain Research, vol 5, pp 135-146.
- Lechan RM, Alpert LC, Jackson IMD. 1976 Synthesis of luteinising hormone releasing factor and thyrotropin-releasing factor in glutamate-lesioned mice. Nature 264:463-465.
- Broadwell RD, Brightman MW. 1976 Entry of peroxidase into neurons of the central and peripheral nervous systems from extracerebral and cerebral blood. J Comp Neurol 166:257-284.
- Lechan RM, Nestler JL, Jacobson S, Reichlin S. 1980 The hypothalamic tuberoinfundibular system of the rat as demonstrated by horseradish peroxidase (HRP) microiontophoresis. Brain Research 195:13-27.
- Wiegand SJ, Price JL. 1980 The cells of the afferent fibers to the median eminence in the rat. J Comp Neurol 192:1-19.
- Lechan RM, Nestler JL, Jacobson S. 1982 The tuberoinfundibular system of the rat as demonstrated by immunohistochemical localization of retrogradely transported wheat germ agglutinin (WGA) from the median eminence. Brain Research 245:1-15.
- Dahlstrom A, Fuxe K. 1964 Evidence for the existence of monoamine-containing neurons in the central nervous system. I. Demonstration of monoamine in the cell bodies of brain stem neurons. Acta Physiol Scand, Suppl, 232:1-55.
- Niimi M, Takahara J, Sato M, Kawanishi K. 1989 Sites of origin of growth hormone-releasing factor-containing neurons projecting to the stalk-median eminence of the rat. Peptides 10:605-608.
- Niimi M, Takahara J, Sato M, Kawanishi K. 1992 Identification of dopamine and growth hormone-releasing factor-containing neurons projecting to the median eminence of the rat by combined retrograde tracing and immunohistochemistry. Neuroendocrinology 55:92-96.
- Merchenthaler I, Lennard DE. 1991 The hypophysiotropic neurotensin-immunoreactive neuronal system of the rat brain. Endocrinology 129:2875-2880.
- Niimi M, Takahara J, Sato M, Kawanishi K. 1991 Neurotensin and growth hormone-releasing factor-containing neurons projecting to the median eminence of the rat: A combined retrograde tracing and immunohistochemical study. Neurosci Letters 133:183-186.
- Takatsu Y, Matsumoto H, Ohtaki T, Kumano S, Kitada C, Onda H, Nishimura O, Fujino M. 2001 Distribution of galanin-like peptide in rat brain. Endocrinology 142: 1626-1634.
- Merchenthaler I. 1992 Enkephalin-immunoreactive neurons in the parvicellular subdivisions of the paraventricular nucleus project to the external zone of the median eminence. J Comp Neurol 326:112-120.
- Ishikawa K, Taniguchi Y, Kurosumi K, Suzuki M, Shinoda M. 1987 Immunohistochemical identification of somatostatin-containing neurons projecting to the median eminence of the rat. Endocrinology 121:94-97.
- Swanson LW, Kuypers HGJM. 1980 The paraventricular nucleus of the hypothalamus: Cytoarchitectonic subdivisions and organization of projections to the pituitary, dorsal vagal complex, and spinal cord as demonstrated by retrograde fluorescence double-labeling methods. J Comp Neurol 194:555-570.
- Kawano H, Tsuruo Y, Bando H, Daikoku S. Hypophysiotrophic TRH-producing neurons identified by combining immunohistochemistry for pro-TRH and retrograde tracing. 1991 J Comp Neurol 307:531-538.
- Niimi M, Takahara, Hashimoto K, Kawanishi K. 1988 Immunohistochemical identification of corticotropin releasing factor-containing neurons projecting to the stalk-median eminence of the rat. Peptides 9:589-593.
- Kawano H, Daikoku S, Shibasaki T. 1988 CRF-containing neuron systems in the rat hypothalamus: retrograde tracing and immunohistochemical studies. J Comp Neurol 272:260-268.
- Dalcik H, Phelps J. 1993 Median eminence-afferent vasoactive intestinal peptide (VIP) neurons in the hypothalamus: localization by simultaneous tract tracing and immunocytochemistry. Peptides 14:1059-1066.
- Merchenthaler I, Setalo G, Csontos C, Petrusz P, Flerko B, Negro-Vilar A. 1989 Combined retrograde tracing and immunocytochemical identification of luteinizing hormone-releasing hormone-and somatostatin-containing neurons projecting to the median eminence of the rat. Endocrinology 125:2812-2821.
- Kawano H, Tsuruo Y, Bando H, Daikoku S. 1991 Hypophysiotropic TRH-producing neurons identified by combining immunohistochemistry for pro-TRH and retrograde tracing. J Comp Neurol 307: 531-538.
- Fekete C, Mihaly E, Luo L-G, Kelly J, Clausen JT, Mao QF, Rand WM, Moss LG, Kuhar M, Emerson CH, Jackson IMD, Lechan RM. 2000 Association of cocaine- and amphetamine-regulated transcript-immunoreactive elements with thyrotropin-releasing hormone-synthesizing neurons in the hypothalamic paraventricular nucleus and its role in the regulation of the hypothalamic-pituitary-thyroid axis during fasting. J Neurosci 20: 9224-9234.
- Silverman AJ, Jhamandas J, Renaud LP. 1987 Localization of luteinizing hormone-releasing hormone (LHRH) neurons that project to the median eminence. J Neurosci 7:2312-2319.
- Goldsmith PC, Thind KK, Song T. 1990 Location of the neuroendocrine gonadotropin-releasing hormone neurons in the monkey hypothalamus by retrograde tracing and immunostaining. J Neuroendocrinology 2:157-168.
- Rugarli EI, Ballabio A. 1993 Kallmann syndrome, from genetics to neurobioloogy. JAMA 270:2713-2716.
- Merchenthaler I. 1991 Neurons with access to the general circulation in the central nervous system of the rat: A retrograde tracing study with fluoro-gold. Neuroscience 44:655-662.
- Palkovits M, Leranth Cs, Zaborszky L, Brownstein MJ. 1977 Electron microscopic evidence of direct neuronal connections from the lower brain stem to the median eminence. Brain Res 136:339-344.
- Reeves WB, Andreoli TE. 1992 The posterior pituitary and water metabolism; Wilson, JD, Foster DW (eds); Williams Textbook of Endocrinology, W B Saunders Co, pp 311-356.
- Vandesande F, Dierickx K. 1975 Identification of vasopressin-producing and of the oxytocin-producing neurons in the hypothalamic magnocellular neurosecretory system of the rat. Cell Tissue Res 164:153-162.
- Stillman MA, Recht LD, Rosario SL, Seif SM, Robinson AG, Zimmerman EA. 1977 The effects of adrenalectomy and glucocorticoid replacement on vasopressin and vasopressin-neurophysin in the zona externa of the median eminence of the rat. Endocrinology 101:42-49.
- Whitnall HM, Smyth D, Gainer H. 1987 Vasopressin coexists in half of the corticotropin-releasing factor axons present in the external zone of the median eminence in normal rats. Neuroendocrinology 45:420-424.
- Raff H. 1993 Interactions between neurohypophysial hormones and the ACTH-adrenocortical axis. Ann N Y Acad Sci 689:411-425.
- Plotsky PM, Bruhn TO, Vale W. 1985 Hypophysiotropic regulation of adrenocorticotropin secretion in response to insulin-induced hypoglycemia. Endocrinology 117:323-329.
- Pow DV, Morris JF. 1989 Dendrites of hypothalamic magnocellular neurons release neurohypophysial peptides by exocytosis. Neuroscience 32:435-439
- Theodosis DT, Bonfanti L, Olive S, Rougon G, Poulain DA. 1994 Adhesion molecules and structural plasticity of the adult hypothalamo-neurohypophysial system. Psychoneuroendocrinology 19: 455-462.
- Theodosis DT. 2002 Oxytocin-secreting neurons: A physiological model of morphological neuronal and dglial plasticity in the adult hypothalamus. Front Neuroendo 23: 101-135
- Morris JF, Pow DV. 1993 New anatomical insights into the inputs and outputs from hypothalamic magnocellular neurons. Ann N Y Acad Sci 689:16-33.
- Chowdrey HS, Lightman SL. 1993 Role of central amino acids and peptide-mediated pathways in neurohypophysial hormone release. Ann N Y Acad Sci 689:183-193.
- Mikkelsen JD, Schmidt P, Sheikh SP, Larsen PJ. 1992 Non-vasopressinergic, non-oxytocinergic neuropeptides in the rat hypothalamo-neurohypophyseal tract: Experimental immunohistochemical studies. Progress in Brain Res 91:367-371.
- Nakamura S, Naruse M, Naruse K, Shioda S, Nakai Y, Uemura H. 1993 Colocalization of immunoreactive endothelin-1 and neurohypophysial hormones in the axons of the neural lobe of the rat pituitary. Endocrinology 32:530-533.
- Brown CH. 2016.Magnocellular neurons and posterior pituitary function, Compr Physiol 6: 1701-1741.
- Stopa EG, LeBlanc VK, Hill DH, Anthony ELP. 1993 A general overview of the anatomy of the neurohypophysis. Ann N Y Acad Sci 689:6-15.
- Mohr E, Fehr S, Richter D. 1991 Axonal transport of neuropeptide encoding mRNAs within the hypothalamo-hypophyseal tract of rats. EMBO J 10:2419-2424.
- Skutella T, Probst JC, Blanco E, Jirikowski GF. 1994 Localization of tyrosine hydroxylase mRNA in the axons of the hypothalamo-neurohypophysial system. Mol Brain Res 23:179-184.
- Moos F, Richard P. 1982 Excitatory effect of dopamine on oxytocin and vasopressin reflex releases in the rat. Brain Res 241:249-260.
- Larsen PJ, Jukes KE, Chowdrey HS, Lightman SL, Jessop DS. 1994 Neuropeptide-Y potentiates the secretion of vasopressin from the neurointermediate lobe of the rat pituitary gland. Endocrinology 134:1635-1639.
- Larsen PJ, Sheikh SP, Mikkelsen JD. 1992 Osmotic regulation of neuropeptide Y and its binding sites in the magnocellular hypothalamo-neurohypophysial pathway. Brain Res 573:181-189.
- Gonzalez AM, Logan A, Ying W, Lappi DA, Berry M, Baird A. 1994 Fibroblast growth factor in the hypothalamic-pituitary axis: Differential expression of fibroblast growth factor-2 and a high affinity receptor. Endocrinology 134:2289-2297.
- Fagin KD, Wiener SG, Dallman MF. 1985 ACTH and corticosterone secretion in rats following removal of the neurointermediate lobe of the pituitary gland. Neuroendocrinology 40:352-362.
- Murai I, Ben-Jonathan N. 1987 Posterior pituitary lobectomy abolishes the suckling-induced rise in prolactin: Evidence for a prolactin-releasing factor in posterior pituitary. Endocrinology 121:205-211.
- Murai I, Ben-Jonathan N. 1990 Acute stimulation of prolactin release by estradiol: Mediation by the posterior pituitary. Endocrinology 126:3179-3184.
- Lechan RM, Arkun K, Toni R. 2019. Pituitary anatomy and development, in Prolactin Disorders, NA Tritos, A Klibanski, Eds, Humana Press: 11-53.
- Saeki N, Sunami K, Kubota M, Murai H, Takanashi J, Iuchi T, Yamaura A. 2001 Heavily T2-weighted MR imaging of white matter tracts in the hypothalamus: normal and pathologic demonstrations. AJNR 22: 1468-1475.
- Anderson E., Haymaker W. 1974 Breakthroughs in hypothalamic and pituitary research. Prog. Bran Res. 41: 1-60
- Kuhlenbeck H. 1973 The central nervous system of vertebrates. Overall morphologic pattern. Vol 3, Karger, p. 717.
- Haymaker W. 1969 Blood supply of the human hypothalamus, in Haymaker W, Anderson E, Nauta WJH (eds): The Hypothalamus. Charles C. Thomas, pp. 210-218.
- Page RB. 1986 The pituitary portal system, in Ganten D., Pfaff D. (eds), Morphology of the Hypothalamus and its connections, Springer-Verlag, pp 1-48.
- Szentagothai J. Flerko B, Mess B, Halasz B. 1972 Hypothalamic control of the anterior pituitary. An experimental-morphological study., 3 ed, Akadémiai Kiaidò, pp 87-92.
- Popa G., Fielding U. 1930 A portal circulation from the pituitary to the hypothalamic region. J. Anat. 65: 88-91.
- Fuxe K, Agnati LF, Harfstrand A, Cintra A, Aronsson M, Zoli M Gustafsson J-A. 1988 Principles for the hormone regulation of wiring transmission and volume transmission in the central nervous system, in Ganten D., Pfaff D. (eds), Neuroendocrinology of Mood, Springer-Verlag, pp 1-54.
- Iliff JJ, Wang M, Liao Y, Plogg BA, Peng W, Gundersen GA, Benveniste H, Vates GE, Deane R, Goldman SA, Nagelhus EA, Nedergaard M.2012A paravascular pathway facilitates CSF flow through the brain parenchyma and the clearance of interstitial solutes, including amyloid b. Sci Transl Med 4:147ra111.
- Rennels ML, Gregory TF, Blaumanis OR, Fujimoto K, Grady PA. 1985 Evidence for a ‘‘paravascular’’ fluid circulation in the mammalian central nervous system, provided by the rapid distribution of tracer protein throughout the brain from the subarachnoid space. Brain Res 326:47- 63.
- Jessen NA, Munk AS, Lundgaard I, Nedergaard M 2015 The Glymphatic system: A beginner's guide Neurochem Res. 40:2583-2599.
- Johnston M, Zakharov A, Papaiconomou C, Salmasi G, Armstrong D. 2004 Evidence of connections between cerebrospinal fluid and nasal lymphatic vessels in humans, non-human primates and other mammalian species. Cerebrospinal Fluid Res 1:2. doi:10.1186/ 1743-8454-1-2
- Jiang Q, Zhang L, Ding G, Davoodi-Bojd E, Li Q, Li L, Sadry N, Nedergaard M, Chopp M, Zhang Z2016 Impairment of the glymphaticsystem after diabetes. J Cereb Blood Flow Metab. 2016 pii: 0271678X16654702. [Epub ahead of print]
- Lechan R M, Fekete C, Toni R 2009 Thyroid Hormone in Neural Tissue. In: Donald W. Pfaff, Arthur P. Arnold, Anne M. Etgen, Susan E. Fahrbach and Robert T. Rubin (Eds), Hormones, Brain and Behavior, 2nd edition, Vol 2. San Diego: Academic Press; 2009. pp. 1289-1328.
- Agnati LF, Genedani S, Lenzi PL, Leo G, Mora F, Ferre S, Fuxe K 2005 Energy gradients for the homeostatic control of brain ECF composition and for VT signal migration: introduction of the tide hypothesis. J Neural Transm112:45–63
- Mascagni P.1787 De lymphaticis profundis capitis et colli In: Vasorum lymphaticorum corporis humani historia et ichonographia. Pars Prima Sectio VII, Art. VI, Siena: Pazzini Carli, pp. 63-64.
- Aspelund A, Antila S, Proulx ST, Karlsen TV, Karaman S, Detmar M, Wiig H, Alitalo K. 2015 A dural lymphatic vascular system that drains brain interstitial fluid and macromolecules. J Exp Med 212, 991–999
- Louveau A, Smirnov I, Keyes TJ, Eccles JD, Rouhani SJ, Peske JD, Derecki NC, Castle D, Mandell JW, Lee KS, Harris TH, Kipnis J.2015 Structural and functional features of central nervous system lymphatic vessels. Nature 523, 337–341.
220 Bucchieri F, Farina F, Zummo G, Cappello F. 2015 Lymphatic vessels of the dura mater: a new discovery? J Anat. 227:702-703.
- Iliff JJ, Goldman SA, Nedergaard M. 2015 Implications of the discovery of brain lymphatic pathways. Lancet Neurol. 10:977-999.
- Cseer HF, Knopf PM 1992 Cervical lymphatics, the blood-brain barrier and the immunoreactivity of the brain: new view. Immunology Today 13:508-512.
- Lécuyer MA, Kebir H, Prat A. 2016 Glial influences on BBB functions and molecular players in immune cell trafficking. Biochim Biophys Acta.1862:472-82.
- Dyess EM, Segerson TP, Liposits Z, Paull WK, Kaplan MM, WU P. Jackson IMD, Lechan RM. 1988 Triiodothyronine exerts direct cell-specific regulation of thyrotropin-releasing hormone gene expression in the hypothalamic paraventricular nucleus. Endocrinology123:2291-2297.
- Lechan R.M., Kakucska I. 1992 Feedback regulation of thyrotropin-releasing hormone gene expression by thyroid hormone in the hypothalamic paraventricular nucleus, Functional Anatomy of the Neuroendocrine Hypothalamus, Wiley, Chichester, Ciba Foundation Symposium 168, pp 144-164.
- Tannenbaum G.S., Martin JB. 1976 Evidence for an endogenous ultradian rhythm governing growth hormone secretion in the rat. Endocrinology 1976; 98:2255-2257.
- Yamauchi N, Shibasaki T, Ling N, Demura H. In vitro release of growth hormone-releasing factor (GRF) from the hypothalamus: somatostatin inhibits GRH release. Regul Pept 1991, 33: 71-78;de los Frailes MT, Cacicedo L, Fernandez G, et al, Role of locally produced growth-hormone-releasing factor in somatostatin regulation by fetal rat brain cells in culture, Neuroendocrinology 1992, 55: 221-229 ).
- Sato M, Frohman LA. Differential effects of central and peripheral administration of growth hormone (GH) and insulin-like growth factor on hypothalamic GH-releasing hormone and somatostatin gene expression in GH-deficiency dward rats, Endocrinology 1993, 133: 793-7990
- Epelbaum J. 1992 Intrahypothalamic neurohormonal interactions in the control of growth hormone secretion. Functional Anatomy of the Neuroendocrine Hypothalamus, Wiley, Chichester, Ciba Foundation Symposium 168, pp 54-68.
- Minami S, Kamegai J, Sugihara H et al. Growth hormone inhibits its own secretion by acting on the hypothalamus through its receptors on neuropeptide Y neurons in the arcuate nucleus and somatostatin neurons in the periventricular nucleus. Endocr J, 1998, 45(suppl): S19-S26)
- Casanueva FF, Villanueva L, Cabranes JA, Cabezas-Cerrato J, Fernandez-Cruz A. 1984 Cholinergic mediation of growth hormone Endocrinol Metab 59: 526-530.
- Cuttler L. 1996 The regulation of growth hormone secretion, End and Metab Clin. NA 25: 541-571.
- Nemeroff CB, Mason GA, Bissette G, Parks R, Schwarcz DA. 1985 Effects of intra-hypothalamic-injection of quinolinic acid on anterior pituitary hormone secretion in the unanesthetized rat. Neuroendocrinology 41: 332-337.
- Acs Z, Lonart G, Makara GB. 1990 Role of hypothalamic factors (growth-hormone-releasing hormone and gamma-aminobutyric acid) in the regulation of growth hormone secretion in the neonatal and adult rat. J. Neuroendocrinol. 52: 156-160.
- Kiss J, Csaba Z, Csaki A. Halasz B. 2006 Glutamatergic innervation of growth hormone-releasing hormone-containing neurons in the hypothalamic arcuate nucleus and somatostatin-containing neurons in the anterior periventricular nucleus of the rat. Brain Res Bull 70: 278-288.
- Radcliff RP, Lookingland KJ, Chapin LT, Tucker HA. 2001 Pituitary adenylate cyclase-activating polypeptide induces secretion of growth hormone in cattle. Domest Anim Endocrinol 2: 187-196.
- Adams BA, Gray SL, Isaac ER, Bianco AC, Vidal-Puig AJ, Sherwood NM. 2008 Feeding and metabolism in mice lacking pituitary adenylate cycolase-activating polypeptide. Endocrinology 149: 1571-1580.
- Anderson LL, Scanes CG. 2012 Nanobiology and physiology of growth hormone secretion. , Exp Biol Med 237: 126-142.
- Smith RG, Van der Ploeg LH, Howard AD, Feighner SD, Cheng K, Hickey GJ, Wyvratt MJ Jr, Fisher MH, Nargund RP, Patchett AA. 1997 Peptidomimetic regulation of growth hormone secretion. Endocr Rev 18: 621-645.
- Kojima H, Hosoda H, Date Y, Nakazato M, Matsuo H, Kangawa K. 1999 Ghrelin is a growth-hormone-releasing acylated peptide from stomach. Nature 402: 656-660.
- Muller et al. Gherelin. 2015 Mol Metab, 4: 437-460.
- Muccioli G, Tschop M, Tapotti M, Deghenghi R, Heiman M, Ghigo E. 2002 Neuroendocrine and peripheral activities of ghrelin: implications in metabolism and obesity. Eu J Pharmacol 440: 235-254.
- Grouselle D, Chaillou E, Caraty A, Bluet-Pajot MT, Zizzari P, Tillet Y, Epelbaum J. 2008 Pulsatile cerebrospinal fluid and plasma ghrelin in relation to growth hormone secretion and food intake in the sheep.J Neuroendocrinol 20: 1138-1146.
- Tschop M, Flora DB, Mayer JP, Heiman ML. 2002 Hypophysectomy prevents sghrelin-induced adiposity and increases gastric ghrelin secretion in rats. Obesity Res 10: 991-999.
- Steyn FJ, Tolle V, Chen C, Epelbaum J. Neuroendocrine regulation of growth hormone ssecretion. Compr Physiol 2016 6: 687-735.)
- Hillard CJ, Beatka M, Sarvaido J. Endocannabinoid signaling and the hypoyhalamic-pituitary-adrenal axis, Compr Physiol 2016, 7:1-15.
- Sawchenko PE, Swanson LW. 1981 Central noradrenergic pathways for the integration of hypothalamic neuroendocrine and autonomic responses. Science 214:685-687.
- Sawchenko PE, Swanson LW, Grzanna R, Howe PRC, Polack J, Bloom SR. 1985 Co-localization of neuropeptide Y-immunoreactivity in brainstem catecholaminergic neurons that project to the paraventricular nucleus of the hypothalamus. J Comp Neurol 249. Plotsky PM, Kjaer A, Sutton SW, Sawchenko PE, Vale W. 1991 Central activin administration modulates corticotropin-releasing hormone and adrenocorticotropin secretion. Endocrinology128: 2520-2525.
- Sarkar S, Fekete C, Legradi G, Lechan RM. 2003 Glucagon like peptide-1(7-36) amide (GLP-1) nerve terminals densely innervate corticotropin-releasing hormone neurons in the hypothalamic paraventricular nucleus. Brain Res 985: 163-168
- Liposits Zs, Sherman D, Phelix C, Paull WK. 1986 Adrenergic innervation of corticotropin releasing factor (CRF)-synthesizing neurons in the hypothalamic paraventricular nucleus in the rat. Histochemistry 84:201-205.
- Liposits Zs, Sievers L, Paull WK. 1988 Neuropeptide-Y and ACTH-immunoreactive innervation of corticotropin releasing factor (CRF)-synthesizing neurons in the hypothalamus of the rat. Histochemistry 88:227-234.
- Saper CB, Lowey AD, Swanson LW, Cowan WM. 1976 Direct hypothalamo-autonomic connections. Brain Res 117: 305-312.
- Sawchenko PE, Li H-Y, Ericsson A. 2000 Circuits and mechanisms governing hypothalamic responses to stress: a tale of two paradigms. Progress in Brain Res 122: 61-77.
- Besedovsky HO, Del Rey A. 1996 Immune-neuro-endocrine interactions: facts and hypotheses. Endocr Rev 17: 64-102.
- Ericsson A, Kovacs K, Sawchenko PE. 1994 A functional anatomical analysis of central pathways subserving the effects of interleukin-1 on stress-related neuroendocrine neurons. J Neurosci 14: 897-913.
- Rivest S, Lacroix S, Vallieres L, Nadeau S, Zhang J, Laflamme N. 2000 How the blood talks to the brain parenchyma and the paraventricular nucleus of the hypothalamus during systemic inflammatory and infectious stimuli. Proc Soc Exp Biol Med 223: 22-28.
- Ericsson A, Arias C, Sawchenko PE. 1997 Evidence for an intramedullary prostaglandin-dependent mechanism in the activtion of stress-related neuroendocrine circuitry by intravenous interleukin-1. J Neurosci 17: 7166-7179.
- Li H-Y, Ericsson A, Sawchenko PE. 1996 Distinct mechanisms underlie activation of hypothalamic neurosecretory neurons and their medullary catecholaminergic afferents in categorically different stress paradigms. Proc Natl Acad Sci, USA 93: 2359-2364.
- Herman JP, Cullinan WE, Young EA, Akil H, Watson SJ. 1992 Selective forebrain fiber tract lesions implicate ventral hippocampal structures in tonic regulation of paraventricular nucleus corticotropin-releasing hormone (CRH) and arginine vasopressin (AVP) mRNA expression. Brain Res 592:228-238.
- Herman JP, Cullinan WE, Watson SJ. 1994 Involvement of the bed nucleus of the stria terminalis in tonic regulation of paraventricular hypothalamic CRH and AVP mRNA expression. J Neuroendocrinology 6:433-442.
- Tuchelt H, Dekker K, Bahr V, Oelkers W. 2000 Dose-response relationship between plasma ACTH and serum cortisol in the insulin-hypoglycaemia test in 25 healthy subjects and 109 patients with pituitary disease, Clin Endocrinol 53: 301-307.
- Dimitrov EL, DeJoseph MR, Brownfield MS, Urban JH. 2007 Involvement of neuropeptide YY1 receptors in the regulation of neuroendocrine corticotropin-releasing hormone neuronal activity. Endocrinology 148: 3666-3673.
- Levy BH, Tasker JG. 2012 Synaptic regulation of the hypothalamic-pituitary-adrenal axis and its modulation by glucocorticoids and stress. Front Cell Neurosci 6: 1-13.
- Singru PS, Sanchez E, Acharya R, Fekete C, Lechan RM. 2008 Mitogen-activated protein kinase contributes to lipopolysaccharide-induced activation of corticotropin-releasing hormone synthesizing neurons in the hypothalamic paraventricular nucleus. Endocrinology 149: 2283-2292.
- Khan AM, Watts AG 2004 Intravenous 2-deoxy-D-glucose injection rapidly elevates levels of the phosphorylated forms of p44/42 mitogen-activated protein kinases (extracellularly regulated kinases 1/2) in rat hypothalamic parvicellular paraventricular neurons. Endocrinology 145:351-359.
- Sasaguri K, Kikuchi M, Hori N, Yuyama N, Onozuka M, Sato S. 2005 Suppression of stress immobilization-induced phosphorylation of ERK 1/2 by biting in the rat hypothalamic paraventricular nucleus. Neurosci Lett 383:160-164
- Abel ED, Ahima RS, Boets ME et al. 2001. Critical role for thyroid hormone receptor beta 2 in the regulation of paraventricular thyrotropin-releaing hormone n;eurons. J Clin Invest 107:1017-1023.
- Lechan RM, Kakucska I. 1992 Feedbsck regulation of thyrotropin-releasing hormone gene expressionby thyroid hormone in the hypothalamic paraventricular nucleus. Ciba Found Symp 168: 144-158; discussion 158-164.
- Fekete C, Lechan RM.2014. Central regulation of hypothalamic-pituitary-thyroid axis under physiological and pathophysiological conditions. Endocr Rev 35:159-194.
- Farkas, E., E. Varga, B. Kovacs, A. Szilvasy-Szabo, A. Cote-Velez, Z. Peterfi, M. Matziari, M. Toth, D. Zelena, Z. Mezricky, A. Kadar, D. Kovari, M. Watanabe, M. Kano, K. Mackie, B. Rozsa, Y. Ruska, B. Toth, Z. Mate, F. Erdelvi, G. Szabo, B. Gereben, R. M. Lechan, J. L. Charli, P. Joseph-Bravo and C. Fekete. 2020. A glial-neuronal circuit in the median eminence regulates thyrotropin-releasing hormone-release via the endocannabinoid system. iScience 23(3): 100921.
- Sanchez E, Vargas MA, Singru PS, Pascual I, Romero F, Fekete C, Charli JL, Lechan RM. 2009. Tanycyte pyroglutamyl peptidase II contributes to regulation of the hypothalamic-pituitary-thyroid axis through glial-axonal associations in the median eminence. Endocrinology 150: 2283-2291.
- Blake NG, Eckland JA, Foster OJF, Lightman SL. 1991 Inhibition of hypothalamic thyrotropin-releasing hormone messenger ribonucleic acid during food deprivation. Endocrinology 129: 2714-2718,
- Legradi G, Emerson CH, Ahima RS, Flier JS, Lechan RM. 1997 Leptin prevents fasting-induced suppression of prothyrotropin-releasing hormone messenger ribonucleic acid in neurons of the hypothalamic paraventricular nucleus. Endocrinology 138: 2569-2576.
- Rondeel JMM, Heide R, deGreef WJ, van Toor H, van Haasteren GAC, Klootwijk W, Visser TH. 1992 Effect of starvation and subsequent refeeding on thyroid function and release of hypothalamic thyrotropin-releasing hormone. Neuroendocrinology 56: 348-353.
- Harris RC, Fang S-L, Azizi F, Lipworth L, Vagenakis AG, Braverman LE. 1978 Effect of starvation on hypothalamic-pituitary-thyroid function in the rat. Metabolism 27: 1074-1083.
- Flier JS, Harris M, Hollenberg AN. 2000 Leptin, nutrition, and the thyroid: the why, the wherefore, and the siring. J Clin Invest 105: 859-861.
- Fekete C, Legradi G, Mihaly E, Huang Q-H, Tatro JB, Rand WM, Emerson CH, Lechan RM. 2000 α-Melanocyte stimulating hormone is contained in nerve terminals innervating thyrotropin-releasing hormone-synthesizing neurons in the hypothalamic paraventricular nucleus and prevents fasting induced suppression of prothyrotropin-releasing hormone gene expression. J Neurosci 20: 1550-1558.
- Fekete C, Kelly J, Mihaly E, Sarkar S, Rand WM, Legradi G, Emerson CH, Lechan RM. 2001 Neuropeptide Y has a central inhibitory action on the hypothalamic-pituitary-thyroid axis. Endocrinology 142: 2606-2613.
- Fekete C, Sarkar S, Rand WM, Harney JW, Emerson CH, Bianco AC, Lechan RM. 2002 Agouti related protein (AGRP) has a central inhibitory action on the hypothalamic-pituitary-thyroid (HPT) axis; comparisons between the effect of AGRP and neuropeptide Y (NPY) on energy homeostasis and the HPT axis. Endocrinology 143: 3846-3853.
- Toni R, Jackson IMD, Lechan RM. 1990 Neuropeptide Y-immunoreactive innervation of thyrotropin-releasing hormone-synthesizing neurons in the rat hypothalamic paraventricular nucleus. Endocrinology126:2444-2453.
282 Legradi G, Lechan RM. 1998 The arcuate nucleus is the major source for neuropeptide Y-innervation of thyrotropin-releasing hormone neurons in the hypothalamic paraventricular nucleus. Endocrinology 139: 3262-3270.
- Legradi G, Lechan RM. 1999 Agouti-related protein containing nerve terminals innervate thyrotropin-releasing hormoneneurons in the hypothalamic paraventricular nucleus. Endocrinology 140: 3643-3652.
- Fekete C, Sarkar S, Rand WM, Harney JW, Emerson CH, Bianco AC, Beck-Sickinger A, Lechan RM. 2002 Neuropeptide Y1 and Y5 receptors mediate the effects of NPY on the hypothalamic-ppituitary-thyroid (HPT) axis. Endocrinology 143: 4513-4519.
- Ollmann MM, Wilson BD, Yang YK, Kerns JA, Barsh GS, Kent SBH, Gantz I, Barsh GF. 1997 Antagonism of central melanocortin receptors in vitro and in vivo by agouti-related protein, Science 278: 135-138.
- Ahima RS, Saper CB, Flier JS, Elmquist JK. 2000 Lepin regulation of neuroendocrine systems, Front Neuroendocrinology 21: 263-307.
- De Groot. 1999 Dangerous dogmas in medicine: the nonthyroidal illness syndrome. J Clin Endocrinol Metab 84: 151-164.
- Sergeyev V, Broberger C, Hokfelt T, 2001 Effect of LPS administration on the expression of POMC, NPY, galanin, CART and MCH mRNAs in the rat hypothalamus, Mol Brain Res 90: 93-100.
- Francis J, MohanKumar PS, MohanKumarSM, Quandri SK. 1999 Systemic administration of lipopolysaccharide increases plasma leptin levels: blockade by soluble interleukin-1 receptor. Endocrine 10: 291-295.
- Tapia-Arancibia L. Arancibia S, Astier H. 1985 Evidence for a1-adrenergic stimulatory control of in vitro release of immunoreactive thyrotropin-releasing hormone from rat median eminence: In vivo corroboration. Endocrinology 116: 2314-2319.
- Lechan RM, and Fekete C. 2007 Infundibular tanycytes as modulators of neuroendocrine function: hypothetical role in the regulation of the thyroid and gonadal axis. Acta Biomed 78 Suppl 1: 84-98.
- Heuer H, Maier MK, Iden S, Mittag J, Friesema EC, Visser TJ, and Bauer K. 2005 The monocarboxylate transporter 8 linked to human psychomotor retardation is highly expressed in thyroid hormone-sensitive neuron populations. Endocrinology 146: 1701-1606.
- Agnati LF, Zoli, Stromberg MI, and Fuxe K. 1995 Intercellular communication in the brain: wiring versus volume transmission. Neuroscience 69: 711-276.
- Diano S, Naftolin F, Goglia F, Csernus V, and Horvath TL. 1998 Monosynaptic pathway between the arcuate nucleus expressing glial type II iodothyronine 5'-deiodinase mRNA and the median eminence-projective TRH cells of the rat paraventricular nucleus. J Neuroendocrinol 10: 731-472.
- Freitas BC, Gereben B, Castillo M, Kallo I, Zeold A, Eqri P, Liposits Z, Zavacki AM, Maciel RM, Jo S, Singru P, Sanchez E, Lechan RM, Bianco AC. 2010 Paracrine signaling by glial cell-derived triiodothyronine activates neuronal gene expression in the rodent brain and human cells. J Clin Invest 120: 2206-22217.
- Joseph-Bravo P, Jaimes-Hoy L, Uribe R-M, Charli J-L. 2015. 60 years of neuroendocrinology: TRH, the first hypophysiotropic releasing hormone isolated: control of the pituitary-thyroid axis, J Endocrinol 226(2): T85-T100.
- Clark IJ, Cummigs JT. 1982 The temporal relationship between gonadotropin-releasing hormone (GnRH) and luteinizing hormone (LH) secretion in ovariectomized ewes. Endocrinology 111: 1737-1739.
- Moenter SM, Caraty A, Locatelli A, Karsch FJ. 1991 Pattern of gonadotropin-releasing hormone (GnRH) secretion leading up to ovulation in the ewe: existence of a preovulatory GnRH surge. Endocrinology 129: 1175-1182.
- Johnson ES, Gendrich RL, White WF. 1976 Delay of puberty and inhibition of reproductive processes in the rat by a gonadotropin-releasing hormone agonist analog. Fertil. Steril.27: 853-860.
- Seminara SB, Messager S, Chatzidaki EE, Thresher RR, Acierno JS J., Shagoury JK, Bo-Abbas Y, Kuohung W, Schwinof KM, Hendrick AG, Zahn D, Dixon J, Kaiser UB, Slaugenhaupt SA, Gusella JF, O’Rahily S, Carlton MB, Crowley Jr WF, Aparicio SA, Colledge WH.2003 The GPR54 gene as a regulator of puberty. N Eng J Med. 349: 1614-1627.
- Muir AI, Chamberlain L, Elshourbagy NA, Michalovich D, Moore DJ., Calamari A, Szekeres PG, Sarau HM, Chambers JK, Murdock P.2001 AXOR12, a novel human G protein-coupled receptor, activated by the peptide Kiss-1. J. Biol. Chem 276: 28969-28975.
- Kotani M, Detheux M, Vandenbogaerde A, Communi D, Vanderwinden JM, Le Poul E. 2001 The metastasis suppressor gene KiSS-1 encodes kisspeptins, the natural ligands of the orphan G protein-coupled receptor GPR54. J Biol Chem 276: 34631-34636.
- Ohtaki T, Shintani Y, Honda S, Matsumoto H, Hori A, Kanehashi K, Terao Y, Kumano S, Takatsu Y, Masuda Y.2001 Metastin suppresses the motility and growth of CHO cells transfected with its receptor. Nature 411: 613-617.
- King JC, Tobet SA, Snavley FL, Arimura AA. 1982 LHRH immunopositive cells and their projections to the median eminence and organum vasculosum of the lamina terminalis. J Comp Neurol 209(3):287-300.
- Vazquez-Martinez R, Shorte SL, Boockfor FR, Frawley LS. 2001 Synthronized exocytotic bursts from gonadotropin-releasing hormone-expressing cells: dual control by intrinsic cellular pulsatility and gap junctional commnication. 2001 Endocrinology 142: 2095-2101.
- Nunemaker CS, DeFazio RA, Geusz M., Herzog ED, Pitts GR, Moenter SM. 2001 Long-term recordings of networks of immortalized GnRH neurons reveal episodic patterns of electrical activity. 2001 J. Neurophysiol. 86: 86-93.
- Messager S, Chatzidaki EE, Ma D Hendrick AG. Zahn D, Dixon J, Thresher RR, Malinge I, Lomet D, Carlton MB, Colledge WH, Caraty A, Aparicio SAJR. 2005 Kisspeptin directly stimulates gonadotropin-releasing hormone release via G protein-coupled receptor 54. PNAS 102: 1761-1766.
- Gottsch ML, Cunningham MJ, Smith JT, Popa SM, Acohido BV, Crowley WF, Seminara S, Clifton DK, Steiner RA. 2004Endocrinology 145: 4073-4077.
- Matsui H, Takatsu Y, Kumano S, Matsumoto H, Ohtaki T. 2004Peripheral administration of metastin induces marked gonadotropin release and ovulation in the rat. Biochem. Biophys Res Commun 320: 383-388.
- Navarro VM, Castellano JM, Fernandez-Fernandez R, Barreiro ML, Roa J, Sanchez-Criado JE, Aguilar E, Dieguez CF, Pinilla L, Tena-Sempere M. 2004Developmental and hormonally regulated messenger ribonucleic acid expression of KISS-1 and its putative receptor, GPR54, in rat hypothalamus and potent luteinizing hormone-releasing activity of KISS-1 peptide. Endocrinology 145: 4565-4574.
- Han SK, Gottsch ML, Lee KJ, Popa SM, Smith JT, Jakawich SK, Clifton DK, Steiner RA, Herbison AE. 2005 Activation of gonadotropin-releasing hormone neurons by kisspeptin as a neuroendocrine switch for the onset of puberty. J Neurosci 25: 11349-11356.
312 D’Anglemont de Tassigny X, Fagg LA, Dixon JPC, Day K, Leitch HG, Hendrick AG, Zahn D, Franceschini I, Caraty A, Carlton MBL, Aparicio SAJR, Colledge WH. 2006 Hypogonadotropic hypogonadism in mice lacking a functional Kiss1 gene. PNAS 104: 10714-10719.
- D’Anglemont de Tassigny X, Fagg LA, Carlton MBL, Colledge WH. 2008 Kisspeptin can stimulate gonadotropin-releasing hormone (GnRH) release by a direct action at GnRH nerve termnals. Endocrinology 149: 3926-2932.
- Seminara SB, Dipietro MJ, Ramaswamy S, Crowley Jr WF, Plant TM. 2006 Continuous human metastin 45-54 infusion desensitizes G protein-coupled receptor 54-induced gonadotropin-releasinghormone release monitored indirectly in the juvenile male rhesus monkey (Macaca mulatta): a finding with therapeutic implications. Endocrinology 147: 2122-2126.
- Ramaswamy S, Guerriero KA, Gibbs RB, Plant TM. 2008 Structural interactions between kisspeptin and GnRH neurons in the mediobasal hypothalamus of the male Rhesus monkey (Macaca mulatta) as revealed by double immunofluorescence and confocal microscopy. Endocrinology 149: 4387-4395.
- Smith JT, Cunningham MJ, Rissman EF, Clifton DK, Steiner RA. 2005 Regulation of Kiss 1 gene expression in the brain of the female mouse. Endocrinology 146: 3686-3692.
- Decourt C, Tillet Y, Caraty A, Franceschini I, Briant C. 2008 Kisspeptin immunoreactive neurons in the equine hypothalamus.Interactions with GnRH neuronal system, J. Chem Neuroanat 36:131-137.
- Franceschini I, Lomet D, Cateau M. Delsol G, Tillet Y. Caraty A. 2006 Kisspeptin immunoreactive cells of the ovine preoptic area and arcuate nucleus co-express estrogen receptor alpha. Neuroscience Letters 410: 225-230.
- Dungan HM, Clifton DK, Steiner RA. 2006 Kisspeptin neurons as central processors in the regulation of gonadotropin-releasing hormone secretion. Endocrinology 147: 1154-1158.
- Stephens SB, Tolson KP, Rouse MLI, Poling MC, Hashimoto-Parktya M, Mellon PL, Kauffman AS. 2015. Absent progesterone signaling in kisspeptin neurons disrupts the LH surge and impairs fertility in female mice. Endocrinology 156: 3091-3097.
- Kauffman AS, Gottsch ML, Roa J, Byquist AC, Crown A, Clifton DK, Hoffman GE, Steiner RA, Tena-Sempere M. 2007 Sexual differentiation of Kiss1 gene expression in the brainof the rat. Endocrinology 148: 1774-1783.
- Homma T, Sakakibara M, Yamada S, Kinoshita M, Iwata K, Tomikawa J, Kanazawa T, Matsui H, Takatsu Y, Ohtaki T, Matsumoto H, Uenoyama Y, Maeda K, Tsukamura H. 2009 Significance of neonatal testicular sex steroids to defeminize anteroventral periventricular kisspeptin neurons and the GnRH/LH surge system in male rats. Biol Reprod 81: 1216-1225.
- Lehman MN, Coolen LM, Goodman RL. 2010 Minireview: kisspeptin/neurokinin B/dynorphin (KNDy) cells of the arcuate nucleus: a central node in the control of gonadotropin-releasing hormone secretion. Endocrinology 151: 3479-3489.
- Roa J, Navarro VM, Tena-Sempere M. 2011 Kisspeptins in reproductive biology: consensus knowledge and recent developments. Biol Reprod 85: 650-660.
- Goodman RL, Hileman SM, Nestor CC et al. 2013. Kisspeptin, neurokinin B, and dynorphin act in the arcuate nucleus to control activity of the GnRH pulse generator in ewes. Endocrinology 154: 4259-4269.
- Treen AK, Luo V, Belsham DD. 2016 Phoenixin activates immortalized GnRH and kisspeptin neurons through the novel receptor GPR173. Mol Endocrinol 30: 872-888.
- Quennell JH, Howell CS, Roa J, Augustine RA, Grattan DR, Anderson GM. 2011 Leptin deficiency and diet-induced obesity reduce hypothalamic kisspeptin expression in mice. Endocrinology 152: 1541-1550.
- Ojeda SR, Lomniczi A, Sandau US. 2008 Glial-gonadotrophin hormone (GnRH) neurone interactions in the median eminence and the control of GnRH secretion, J. Neuroendocrinology 20: 732-742.
- Garcia-Segura M, Lorenz B, DonCarols LL. 2008 The role of glia in the hypothalamus: implications for gonadal steroid feedback and reproductive neuroendocrine output. Reproduction 135: 419-429.
- Prevot V., Dehouck B., Poulain P., Beauvillain J-C., Buee-Scherrer V., Bouret S. 2007 Neuronal-glial-endothelial interactions and cell plasticity in the postnatal hypothalamus: implications for the neuroendocrine control of reproduction. Psychoneuroendocrinology 32: S46-S51.
- Sharif A, Baroncini M, Prevot V. 2013. Role of glia in the regulation of gonadotropin-releasing hormone neuronal activity and secretion. Neuroendocrinology 98: 1-15.
- Langub M.C., Watson RE. Jr. 1992 Estrogen receptor-immunoreactive glia, endothelia, and ependyma in guinea pig preoptic area and median eminence: electron microscopy. Endocrinology 130: 364-372.
- Rivier C, Rivier J, Vale W. 1986 Stress-induced inhibition of reproductive functions: role of endogenous corticotropin-releasing factor, Science 231: 607-609.
- Freeman ME, Kanyicska B, Lerant A, Nagy G. 2000 Prolactin: structure, function and regulation of secretion. Physiol Rev 80: 1523-1631.
- Stricker EM, Verbalis JG. Water intake and body fluids, in Fundamental Neuroscience, ch 42, pp 1111-1126, 1999.
- Dunn FL, Brennan RJ, Nelson AE, Robertson GL. 1973 The role of blood osmolality and volume in regulatig vasopressin secretion in the rat. J Clin Invest 52: 3212-3219.
- Shaw S, Marples D. 2002 A rat kidney tubule suspension for the study of vasopressin-induced shuttling of AQP2 water channels. Am J Physiol Renal Physiol. 283: F1160-F1166
- Valenti G, Procino G, Tamma G, Carmosino M, Svelto M. 2005 Minireview: aquaporin 2 trafficking. Endocrinology 146: 5963-5070.
- Osaka T, Kannan H, Kasai M, Inenaga K, Yamashita H. 1990 Osmotic responses of rat paraventricular neurons by pressure ejection method. Brain Res. Bull. 24: 493-497.
- Honda K, Negoro H, Higuchi T, Tadokoro Y. 1987 Activation of neurosecretory cells by osmotic stimulation of anteroventral third ventricle. Am J Physiol. 252: R1039-1045.
- McKinley MJ, Bicknell RJ, Hards D, McAllen RM, Vivas L, Weisinger RS, Oldfield BJ. 1992 Efferent neural pathways of the lamina terminalis subserving osmoregulation. Prog. Brain Res. 91: 395-402.
- Ferguson AV, Renaud LP. 1986 Systemic angiotensin acts at subfornical organ to facilitate activity of neurohypophysial neurons. Am J Physiol 251: R712-R717.
- Liedtke W. 2007 Hydromineral neuroendocrinology: role of TRPV ion channels in sensory transduction of osmotic stimuli in mammals. Exp. Physiol. 92: 507-512.
- Forsling ML, Ingram DL, Stanier MW. 1976 Effects of various ambient temperatures and of heating and cooling the hypothalamus and cervical spinal cord on antidiuretic hormone secretion and urinary osmolality in pigs. J Physiol 257: 673-686.
- Azahan E, Sykes AH. 1980 The effects of ambient temperature on urinary flow and composition in the fowl. J Physiol 304: 389-396.
- Sharif-Naeini R, Ciura S, Bourque CW. 2008 TRPV1 gene required for thermosensory transduction and anticipatory secretion from vasopressin neurons during hyperthermia. Neuron 58: 179-185.
- Hiyama TY, Matsuda S, Fujikawa A, Matsumoto M, Watanabe E, Kajiwara H, Nimura F, Noda M. 2010 Autoimmunity to the sodium-level sensor in the brain causes essential hypernatremia. Neuron 66: 508-522.
- Dohanics J, Linton EA, Lowry PJ, Makara GB. 1990 Osmotic stimulation affects neurohypophysial corticotropin releasing factor-41 content: Effect of dexamethasone. Peptides 11:51-57.
- Oelkers W. 1989 Hyponatremia and inappropriate secretion of vasopressin (antidiuretic hormone) in patients with hypopituitarism. N Engl J Med 321:492-496.
- Kiss JZ, Van Eekelen AM, Reul JMHM, Westphal HM, DeKloet ER. 1985 Glucocorticoid receptor in magnocellular neurosecretory cells. Endocrinology 122:444-449.
- Morris JF, Pow DV. 1993 New anatomical insights into the inputs and outputs from hypothalalmic magnocellular neurons. Ann N Y Acad Sci 689: 16-33.
- Chowdrey HS, Lightman SL. 1993 Role of central amino acids and peptide-mediated pathways in neurohypophysial hormone release. Ann N Y Acad Sci 689: 183-193.
- Hetherington AW, Ranson SW. 1940 Hypothalamic lesions and adiposity in the rat, Anat Rec 78: 149-172
- Reeves AG and Plum F. 1969 Hyperphagia, rage amd dementia accompanying a ventromedial hypothalamic neoplasm. Arch Neurol 20: 616-624.
- Zhang Y, Proenca R, Maffei M, Barone M, Leopold L, Friedman JM. 1994 Positional cloning of the mouse obese gene and its human homologue, Nature 372: 425-432.
- Schwartz MW, Woods SC, Porte D Jr, Seeley RJ, Baskin DG. 2000 Central nervous system control of food intake, Nature 404: 661-671.
- Kalra SP, Dube MG, Pu S, Xu B, Horvath TL, Kalra PS. 1999 Interacting appetite-regulating pathways in the hypothalamic regulation of body weight, Endocr Rev 20: 68-100.
- Barsh GS, Schwartz. 2002 Genetic approaches to studying energy balance: perception and integration, Nature Rev 3: 589-600.
- Gautren L, Elmquist JK. 2011 Sixteen years and counting: an update on leptin in energy balance. J Clin Invest 121: 2087-2093.
- Zhao AZ, Huan J-N, Gupta S, Pal R, Sahu A. 2002 A phosphatidylinositol 3-kinase phosphodiesterase 3B-cyclic AMP pathway in hypothalamic action of leptin on feeding. Nat. Neurosci. 5: 727-728.
- Bates SH, Stearns WH, Dundon TA, Schubert M, Tso AW, Wang Y, Banks AS, Lavery HJ, Haq AK, Maratos-Flier E, Neel BG, Schwartz MW, Myers Jr MG. 2003 STAT3 signaling is required for leptin regulation of energy balance but not reproduction. Nature 421: 856-859.
- Kwon O, Kim KW, Kim M-S. 2016 Leptin signaling pathways in hypothalamic neurons, Cell Mol Life Sci 73: 1457-1477.
- Nejenhuis WAJ, Oosterom J, Adan RAH. 2001 AgRP (83-132) acts as an inverse agonist on the human-melanocortin-4 receptor, Mol Endocrinol 15: 164-171.
- Cowley MA, Smart JL, Rubinstein M, Cerdan MG, Diano S, Horvath TL, Cone RD, Low MJ. 2001 Leptin activates anorexigenic POMC neurons through a neural network in the arcuate nucleus, Nature 411: 480-484.
- Erickson JC, Clegg KE, Palmiter RD. 1996 Sensitivity to leptin and susceptibility to seizures of mice lacking neuropeptide Y, Nature 381: 415-421.
- Huzar D, Lynch CA, Fairchild-Huntress V, Dunmore JH, Fang Q, Berkemeier LR, Gu W, Kesterson RA, Boston BA, Cone RD, Smith FJ, Campfield LA, Burn P, Lee F. 1997 Targeted disruption of the melanocortin-4 receptor results in obesity in mice, Cell 88: 131-141.
- Krude H, Biebermann H, Luck W, Horn R, Brabant G, Gruters A. 1998 Severe early-onset obesity, adrenal insufficiency and red hair pigmentation caused by POMC mutations in humans, Nat Genet 19: 1155-1157.
- Cheetham T, O’Rahilly S. 2000 Dominant and recessive inheritance of morbid obesity associated with melanocortin 4 receptor deficiency, J Clin Invest 106: 271-279.
- Jackson RS, Creemers JW, Ohagi S, Raffin-Sanson ML, Sanders L, Montague CT, Hutton JC, O’Rahilly S. 1997 Obesisty and impaired prohormone processing associated with mutations in the human prohormone convertase 1 gene, Nature Genet 6: 218-220.
- Gruenewald DA, Matsumoto AM. 1991 Age-related decrease in proopiomelanocortin gene expression in the arcuate nucleus of the male rat brain, Neurobiol Aging 12: 113-121.
- Mobbs CV, Bray GA, Atkinson RL, Bartke A, Finach CE, Maratos-Flier E, Crawley JN. Nelson JF. 2001 Neuroendocrine and pharmacological manipulations to assess how caloric restriction increase lifespan, J Gerontology 56: 34-44.
- Wisse BE, Frayo RS, Schwartz MW, Cummings DE. 2001 Reversal of cancer anorexia by blockade of central melanocortin receptors in rats, Endocrinology 142: 3292-3101.
- Marks DL, Ling N, Cone RD. 2001 Role of the central melanocortin system in cachexia, Cancer Res 61: 1432-1438.
- Kuhnen K, Clement S, Wiegand et al. 2018 Proopiomelanocortin deficiency treated with a melanocortih-4 receptor agonist. N Engl J Med 2016; 375: 240-246, Clement H Bibermann IS Farooqi et al, MC4R agonism promotes durable weight loss in patients with leptin receptor deficiency. Nat Med 24: 551-555.
- Sarkar S, Legradi G, Lechan RM. 2002 Intracerebroventricular administration of -melanocyte stimulating hormone increases phosphorylation of CREB in TRH-producing and CRH-producing neurons of the hypothalamic pavaventricular nucleus. Brain Res 945: 50-59.
- Hahn TM, Breininger JF, Baskin DG, Schwartz MW. 1998 Coexpression of Agrp and NPY in fasting-activated hypothalalmic neurons. Nat Neurosci 1: 271-272.
- Spiegelman BM, Flier JS. 2001 Obesity and the regulation of energy balance. Cell 104: 531-543.
- Wirth MM, Olszewski PK, Yu C, Levine AS, Giraudo SQ. 2001 Paraventricular hypothalamic alpha-melanocyte-stimulating hormone and MTII reduce feeding without causing aversive effects. Peptides 22: 129-134.
- Giraudo SQ, Billington CJ, Levine AS. 1998 Feeding effects of hypothalamic injection of melanocortin 4 receptor ligands. Brain Res 809: 302-306.
- Luiten PG, ter Horst GJ, Karst H, Steffens AB. 1985 The course of paraventricular hypothalamic efferents to autonomic structures in medulla and spinal cord. Brain Res 329: 374-378.
- Roeling TA, Veening JG, Peters JP, Vermelis ME, Nieuwenhuys R. 1993 Efferent connections of the hypothalamic “grooming area” in the rat. Neuroscience 56: 199-225.
- Sawchenko PE, Swanson LW. Immunohistochemical identification of neurons in the paraventricular nucleus of the hypothalamus that project to the medulla or to the spinal cord in the rat. J Comp Neurol 1982; 205:260-272.
- Haynes WG, Morgan DA, Djalali A, Sivitz WI, Mark AL. 1999 Interactions between the melanocortin system and leptinin control of sympathetic nerve traffic. Hypertension 33: 542-547.
- Bamshad M, Aoki VT, Adkison MG, Warren WS, Bartness TJ. 1998 Central nervous system origins of the sympathetic nervous system outflow to white adipose tissue. Am J Physiol 275: R291-R299.
- Shah BP, Vong L, Olson DP, Koda S, Krashes MJ, Ye C, Yang Z, Fuller PM, Elmquist JK, Lowell BB.2014 MC4R-expressing glutamatergic neurons in the paraventricular hypothalamus regulate feeding and are synaptically connected to the parabrachial nucleus. Proc Natl Acad Sci USA 111: 13193-13198.
- Carter ME, Soden ME, Zweifel LS, Palmiter RD. 201. Genetic identification of a neural circuit that suppresses appetite.Nature 503: 111-114.
- Blouet C, Jo YH, Li X, Schwaratz GJ. 2009 Mediobasal hypothalamic leucine sensing regulates food intake through activation of a hypothalamus-brainstem circuit. J Neurosci 29: 8302-8311.
- Singru PS, Wittmann G, Farkas E, Zseli G, Fekete C, Lechan RM. 2012 Refeeding-activated glutamatergic neurons in the hypothalamic paraventricular nucleus (PVN) mediate effects of melanocortin signaling in the nucleus tractus solitarius (NTS). Endocrinology 153: 3804-3814.
- Blevins JE, Morton GJ, Williams DL, Caldwell DW, Bastian LS, Wisse BE, Schwartz MW, Baskin DG. 2009 Forebrain melanocortin signaling enhances the hindbrain satiety reponse to CCK-8. Am J Physiol Regul Integr Comp Physiol 296: R476-484.
- Blevins JE, Schwartz MW, Baskin DG. 2004 Evidence that paraventricular nucleus oxytocin neurons link hypothalamic leptin action to caudal brain stem nuclei controlling meal size. Am J Physiol Regul Integr Comp Physiol 287: R97-96.
- Elmquist JK. 2001 Hypothalamic pathways underlying the endocrine, autonomic, and behavioral effects of leptin. Phys Behav 74: 703-708.
- Saper CB. 1985 Organization of cerebral cortical afferent systems in the rat. II. Hypothalamocortical projections. J Comp Neurol 237: 21-46.
- Qu D, Ludwig DS, Gammeltoft S, Piper M, Pelleymounter MA, Cullen MJ, Mathes WF, Przypek R, Kanarek R, Maratos-Flier E. 1996 A role for melanin-concentrating hormone in the central regulation of feeding behaviour, Nature 380: 243-247.
- Shimada M, Tritos NA, Lowell BB, Flier JS, Maratos-Flier E. 1998 Mice lacking melanin-concentrating hormone are hypophagic and lean. Nature 396: 670-674.
- Sakurai T. 2002 Roles of orexins inregulation of feeding and wakefulness. Neuroreport 13: 987-995.
- Krowicki ZK, Burmeister MA, Berthoud HR, Scullion RT, Fuchs K, Hornby PJ. 2002 Orexins in rat dorsal motor nucleus of the vagus potently stimulate gastric motor function. Am J Physiol Gasstrointest Liver Physiol 283: G465-472.
- Elias CF, Lee C, Kelly J, Aschkenasi C, Ahima RS, Couceyro PR, Kuhar MJ, Saper CB, Elmquist JK. 1998 Leptin activates hypothalamic CART neurons projecting to the spinal cord. Neuron 21: 1375-1385.
- Ahima RS, Flier JS. 2000 Leptin. Annu Rev Physiol 62: 413-437.
399 Morton GJ. Niswender KD, Rhodes CJ, Myers MG, Blevins JE, Baskin DG, Schwartz MW. 2003 Arcuate nucleus-specific leptin receptor gene therapy attenuates the obesity phenotype of Koletsky (fa(k)/fa(k)) rats. Endocrinology 144: 2016-2024.
- Coppari R, Ichinose M, Lee CE, Pullen AE, Kenny CD, McGovern RA, Tang V, Liu SM, Ludwig T, Chua SC Jr, Lowell BB, Wlmquist JK. 2005 The hypothalamic arcuate nucleus: a key site for mediating leptin’s effects on glucose homeostasis and locomotor activity. Cell Metab 1: 63-72.
- ter Horst GJ, Luiten PGM. 1986 The projections of the dorsomedial hypothalamic nucleus in the rat. Brain Res Bull 16: 231-248.
- Bellinger LL, Bernardis LL. 2002 The dorsomedial hypothalamic nucleus and its role in ingestive behavior and body weight regulation: lesions learned from lesioning studies. Physiol Behav 76: 431-442.
- Bellinger LL, Bernardis LL, Williams FE. 1984 Suppressionof feeding b y cholecystokinin but not bombesin is attenuated by dorsomedial hypothalamic nuclei lesioned rats. Peptides 5: 547-552.
- Fulwiler CE, Saper CB. 1985 Cholecystokinin-immunoreactive innervation of the ventromedial hypothalamus in the rat: possible substrate for autonomic regulation of feeding. Neurosci Lett 53: 289-296.
- Choi S, Wong LS, Yamat C, Dallman MF. 1998 Hypothalamic ventromedial nuclei amplify circadian rhythms: Do they contain a food-entrained endogenous oscillator? J Neurosci 18: 3843-3852.
- Grill HJ, Schwartz MW, Kaplan JM, Foxhall JS, Breininger J, Baskin DG. 2002 Evidence that the caudal brainstem is a target for the inhibitory effect of leptin on food intake. Endocrinology 143: 239-246.
- Goldstone AP, Mercer JG, Gunn I, Moar KM, Edwards CM, Tossi M, Howard JK, Rasheed S, Turton MD, Small C, Heath MM, O’Shea D, Steere J, Meeran K, Ghatei MA, Hoggard N, Bloom SR. 1997 Leptin interacts with glucagons-like peptide-1 neurons to reduce food intake and body weight in rodents. FEBS Lett 415: 134-138.
- Al-Barazanji KA, Arch JR, Buckingham RE, Tadayyon M. 2000 Central exendin-4 infusion reduces body weight without altering plasma leptin in (fa/fa) Zucker rats. Obes Res 8: 317-323.
- Merchenthaler I, Lane M, Shughrue P. 1999 Distribution of pre-pro-glucagon and glucagons-like peptide-1 receptor messenger RNAs in the rat central nervous system. J Comp Neurol 403: 261-280.
- Turton MD, O’Shea D, Gunn I, Beak SA, Edwards CM, Meeran K, Choi SJ, Taylor GM, Heath MM, Lambert PD, Wilding JP, Smith DM, Ghatei MA, Herbert J, Bloom SSR. 1996 A role for glucagons-like peptide-1 in the central regulation of feeding. Nature 379: 69-72.
- Larsen PJ, Tang-Christensen M, Holst JJ, Orskov C. 1997 Distribution of glucagons-like peptide-1 and other preproglucagon-derived peptides in the rat hypothalamus and brainstem. Neuroscience 77: 257-270.
- McMahon LR, Welllman PJ. 1998 PVN infusion of GLP-1-(7-36) amide suppresses feeding but does not induce aversion or alter locomotion in rats. Am J Physiol 274: R23-R29.
- Hayes MR, Skibicka KP, Leichner TM, Guarnieri DJ, DiLeone RJ, Bence KK, Grill HJ. 2010 Endogenous leptin signaling in the caudal nucleus tractus solitarius and area postrema is required for energy balance regulation. Cell Metab 11: 77-83.
- Hommel JD, Trinko R, Sears RM, Georgescu D, Liu ZW, Gao XB, Thurmon JJ, Marinelli M, DiLeone RJ. 2006 Leptin receptor signaling in midbrain dopamine neurons regulates feeding. Neuron 51: 801-810.
- Naslund E, Hellstrom PM. 2007 Appetite signaling: From gut peptides and enteric nerves to brain. Physiol. Behav. 92: 265-262.
- Suzuki K, Jayasena CN, Bloom SR. 2012 Obesity and appetite control. Exp Diabetes Res, Article ID 824305, 1-19.
- Bauer PV, Hamr SC, Duca FA. 2016. Regulation of energy balance by a gut-brain axis and involvement of the gut microbiota.Cell Mol Life Sci 73: 737-755.
- Murphy KG, Dhillo WS, Bloom SR. 2006 Gut peptides in the regulation of food intake and energy homeostasis. Endocrine Reviews 27: 719-727.
- Schwartz MW, Sipols AJ, Marks JL, Sanacora G, White JD, Scheurink A, Kahn SE, Baskin DG, Woods SC, Figlewicz DP. 1992 Inhibition of hypothalamic neuropeptide Y gene expression by insulin. Endocrinology 130: 3608-3616.
- Woods SC, Seeley RJ, Porte D Jr, Schwartz MW. 1998 Signals that regulate food intake and energy homeostasis. Science 280: 1378-1383.
- Reda TK, Geliebter A, Pi-Sunyer FX. 2002 Amylin, food intake, and obesity. Obes Res 10: 1087-1091.
- Schwartz GJ, McHugh PR, Moran TH. 1993 Gastric loads and cholecysstokinin synergistically stimulate rat gastric vagal afferents. Am J Physiol 265: R872-R876.
- Batterham RL, Cowley MA, Small CJ, Herzog H, Cohen MA, Dakin CL, Wren AM, Brynes AE, Low MJ, Ghatel M, Cone RD, Bloom SR. 2002 Gut hormone PYY3-36 physiologically inhibits food intake. Nature 418: 650-654.
- Abbot CR, Monteiro M, Small CJ, SajediA, Smith KL, Parkinson JR, Ghatei MA, Bloom SR. 2005 The inhibitory effects of peripheral administration of peptide YY (3-36) and glucagon-like peptide-1 on food intake are attenuated by ablation of the vagal-brainstem-hypothalamic pathway. Brain Res. 1044:127-131.
- Baggio LL, Huang Q, Brown TJ, Drucker DJ. 2004 Oxntomodulin and glucagon-like peptide-1 differently regulate murine foodintake and energy expenditure. Gastroenterology 127: 546-558.
- Wynne K, Park AJ, Small CJ, Meeran K, Ghatei MA, Frost GS, Bloom SR. 2006 Oxyntomodulin increases energy expenditure in addition to decreasing energy intake in overweight and obese humans: a randomized controlled trial.Int. J. Obes. 30: 1729-1736.
- Asakawa A, Inui A, Yuzuriha H, Ueno N, Katsuura G, Fujimiya M, Fujino MA, Niijima A, Meguid MM, Kasuga M. 2003. Characterization of the effects of pancreatic plypeptide in the regulation of energy balance. Gastroenterology 124: 1325-1336.
- Cummings DE, Purnell JQ, Frayo RS, Schmidova K, Wisse BE, Weigle DS. 2001 A preprandial rise inplasma ghrelin levels suggests a arole in meal initiation in humans. Diabetes 50: 1714-1719.
- Kamegai J, Tamura H, Shimizu T, Ishii S, Sugihara H, Wakabayashi I. 2001 Chronic central infusion of ghrelin increases hypothalamic neuropeptide Y and agouti-related protein mRNA levels and body weight in rats. Diabetes 50: 2438-2443.
- Williams KW, Elmquist JK. 2012 From neuroanatomy to behavior: central integration of peripheral signals regulating feeding behavior. Nat Neurosci 15: 1530-1355.
- Williams DL, Grill HJ, Cummings DE, Kaplan JM. 2003 Vagotomy dissociates short- and long-term controls of circulating ghrelin. Endocrinology 144: 5184-5187.
- Cummings DE, Clement K, Purnell JQ, Vaisse C, Foster KE, Frayo RS, Schwartz MW, Basdevant A, Weigle DS. 2002 Elevated plasma ghrelin levels in Prader Willi syndrome. Nat Med. 8:643-4.
- Goldstone AP, Thomas EL, Brynes AE, Castroman G, Edwards R, Ghatei MA, Frost G, Holland AJ, Grossman AB, Dorbonits M, Bloom SR, Bell JD. 2004 Elevated fasting plasma ghrelin in Prader-Willi syndrome adults is not solely explained by their reduced visceral adiposity and insulin resistance. J Clin. Endocrinol. Metab. 89: 1718-1726.
- Zhang JV, Ren PG, Avsian-Kretchmer O, Luo GW, Rauch R, Klein C, Hsueh AJ. 2005 Obestatin, a peptide encoded by the ghrelin gene, oposes ghrelin’s effects on food intake. Science 310: 996-999.
- Stengel A, Gobel M, Yakubov I, Wang L, Witcher D, Coskun T, Tache Y, Sachs G, Lambrecht NW. 2008 Identification and characterization of nesfatin-1 immunoreactivity in endocrine cell types of the rat gastric oxyntic mucosa. Endocrinology, in press.
- Oh-I S, Shimizu H, Satoh T, Okada S, Adachi S, Inoue K, Eguchi H, Yamamoto M, Imaki T, Hashimoto K, Tsuchiya T, Monden T, Horiguchi K, Yamada M, Mori M. 2006 Identification of nesfatin-1 as a satiety molecule in the hypothalamus. Nature 443: 709-712.
- Price CJ, Samson WK, Ferguson AV. 2008 Nesfatin-1 inhibits NPY neurons in the arcuate nucleus. Brain Res 1230: 99-106.
- Fort P, Salvert D, Hanriot L, Jego S, Shimizu H, Hashimoto K, Mori M, Luppi PH. 2008 Neuroscience The satiety molecule nesfatin-1 is co-expressed with melanin concentrating hormone in tuberal hyhpothalamic neurons of the rat. Neuroscience 155: 174-181.
- Gavrieli A, Mantzoros CS. 2016. Novel molecules regulating energy homeostasis: physiology and regulation by macronutrient intake and weight loss. Endocrinology and Metabolism 31: 361-372.
- Abraham G, Falcou R, Rozen R, Mandenoff A, Autissier N, Apfelbaum M. 1987 The effects of a constant T3 level and thermoneutrality in diet-induced hyperphagia. Horm Metab Res 19(3): 96-100.
- Syed MA, Thompson MP, Pachucki J, Burmeister LA. 1999 The effect of thyroid hormone on size of fat depots accounts for most of the changes in leptin mRNA and serum levels in the rat. Thyroid 9(5): 503-512.
- Ishii S. Kamegai J, Tamura H, Shimizu T, Sugihara H, Oikawa S. 2003 Hypothalamic neuropeptide Y/Y1 receptor pathway activated by a reduction in circulating leptin, but not by an increase in circulating ghrelin, contributes to hyperphagia associated with triiodothyronine-induced thyrotoxicosis. Neuroendocrinology 78(6): 321-230.
- Luo L, MacLean DB. 2003 Effects of thyroid hormone on food intake, hypothalamic Na/K ATPase activity and ATP content. Brain Res 973(2): 233-239.
444 Kong W M, Martin NM, Smith KL, Gardiner JV, Connoley IP, Stephens DA, Dhillo WS, Ghatei MA, Small CJ, Bloom SR. 2004 Tri-iodothyronine Stimulates Food Intake via the Hypothalamic Ventromedial Nucleus Independent of Changes in Energy Expenditure. Endocrinology 145: 5252-5258.
- Coppla A, Liu ZW, Andrews ZB, Paradis E, Roy MC, Friedman JM, Ricquier D, Richard D, Horvath TL, Gao XB, Diano S. 2007 A central thermogenic-like mechanism in feeding regulation: an interplay between arcuate nucleus T3 and UCP2.Cell Metab. 5: 21-33.
- Puymirat, J. 1992 Thyroid receptors in the rat brain. Prog Neurobiol 39(3): 281-294.
- Lechan RM, Qi Y, Jackson IMD, Mahdavi V. 1994 Identification of thyroid hormone receptor isoforms in thyrotropin-releasing hormone neurons of the hypothalamic paraventricular nucleus. Endocrinology 135(1): 92-100.
- Broedel O, Eravci M, Stoltenburg-Didinger G, Plueckhan H, Fuxius S, Meinhold H, Baumgartner A. 2003 Effects of hyper- and hypothyroidism on thyroid hormone concentrations in regions of the rat brain. Am J Physiol Endocrinol Metab 285(3): E470-E480.
- Campos-Barros A, Musa A, Flechner A, Hessenius C, Gaio U, Meinhold H, Baumgartner A. 1997 Evidence for circadian variations of thyroid hormone concentrations and type II 5'-iodothyronine deiodinase activity in the rat central nervous system. J Neurochem 68(2): 795-803.
- Heine A, Taylor JA, Iwamoto GS, et al. 2000. Increased adipose tissue in male and female estrogen receptor-alpha knockout mice. Proc Natl Acad Sci USA, 97: 12729-12734.
- Xu Y, Nedungadi TP, Zhu L, et al. 2011. Distinct hypothalamic neurons mediate estrogenic effects on energy homeostasis and reproduction. Cell Metab 14: 453-465.
- Kadowaki T, Yamauchi T, Kubota N. 2008 The physiological and pathophysiological role of adiponectin and adiponectin receptors in the peripheral tissues and CNS. FEBS Lett 582: 74-80.
453 Kubota N, Yano W, Kubota T, Yamauchi T, Itoh S, Kumagai H, Kozono H, Takamoto I, Okamoto S, Shiuchi T, Suzuki R, Satoh H, Tsuchida A, Moroi M, Sugi K, Noda T, Ebinuma H, Ueta Y, Kondo T, Araki E, Ezaki O, Nagai R, Tobe K, Terauchi Y, Ueki K, Minokoshi Y, Kadowaki T. 2007 Adiponectin stimulates AMP-activated protein kinase in the hypothalamus and increases food intake. Cell Metab 6: 55-68.
- Lechan RM, Fekete C. 2010 Neuroendocrine and metabolic adaptations in the central nervous system to weight loss that facilitate weight re-gain. Pediatric Obesity: Etiology, Pathogenesis, and Treatment, M. Freemark, Ed, Humana Press/Springer, pp
- Williams KW, Elmquist JK. 2012 From neuroanatomy to behavior: central integration of peripheral signals regulating feeding behavior. Nat Neurosci 15: 1350-1355.
- Piomeilli D. 2003 The molecular logic of endocannabinoids signaling. Nat Rev Neurosci 4: 873-884.
- Wittmann G, Deli L, Kallo I, Hrabovszky E, Watanabe M, Liposits Z, Fekete C. 2007 Distribution of type 1 cannabinoid receptor (CB1)-immunoreactive axons in the mouse hypothalamus. J Comp Neurol 10: 270-279.
- Freund TF, Katona I, Piomelli D. 2003 Role of endogenous cannabinoids in synaptic signaling. Physiol Rev 83: 1017-1066.
- Kola B, Farkas I, Christ-Crain M, Wittmann G, Lolli F, Amin F, Harvey-White J, Liposits Z, Kunos G, Frossman AB, Fekete C, Korbonits M. 2008 The orexigenic effect of ghrelin is mediated through central activationof the endogenous cannabinoid system. PLoS One 3:e1797.
- Jo YH, Chen YJ, Chua SC Jr, Talmage DA, Role LW. 2005 Integration of endocannabinoids and leptin signaling in an appetite-related neural circuit. Neuron 48: 1055-1066.
- Tong Q, Ye CP, Jones JE, Elmquist JK, Lowell BB. 2008 Synaptic release of GABA by AgRP neurons is required for normal regulation of energy balance. Nat Neurosci 11: 998-1000.
- Vong L, Ye C, Yang Z, Choi B, Chua S Jr, Lowell BB. 2011 Leptin action on GABAergic neurons prevents obesity and reduces inhibitory tone to POMC neurons. Neuron 14: 142-154.
- Xu Y, Berglund ED, Sohn JW, Holland WL, Chuang JC, Fukuda M, Rossi J, Williams KW, Jones JE, Zigman JM, Lowell BB, Sherer PE, Elmquist JK. 2010 5-HT2CRs expressed by pro-opiomelanocortin neurons regulate insulin sensitivity in liver. Nat Neurosci 13: 1457-1459.
- Xu Y, Jonwa JE, Kohno S WR. 2008 L 5-HT2CRs expressed by pro-opiomenalocortin neurons regulate energy homeostasis. Neuron 60: 582-589.
- Burke LK, Heisler LK. 2015. 5-hydroxytryptamine medications sfor the treatment of obesity. J Neuroendocrinol 27: 389-398.
- Jarvie BC, Hentges ST. 2012 Expression of GABAergic and glutamatergic phenotypic markers in hypothalamic proopiomelanocortin neurons. J Comp Neurol 520: 3863-3876.
- Fulton S, Pissios P, Manchon RP, Stiles L, Frank L, Pothos EN, Maratos-Flier E, Flier JS. 2006 Leptin regulation of the mesoaccumbens dopamine pathway. Neuron 51: 811-822.
- Kelly AE, Berridge KC. 2002 The neuroscience of natural rewards: relevance to addictive drugs. J Neurosci 22: 3306-3311.
- Geiger BM, Haburcak M, Avena NM, Moyer MC, Hoebel BG, Pothos EN. 2009 Deficits of mesolimbic dopamine neurotransmission in rat dietary obesity. Neuroscience 159: 1193-1199.
- Ritter S, Dinh TT, Li AJ. 2006 Hindbrain catecholamine neurons control multiple glucoregulatory reponses. Physiol. Behav. 89: 490-500.
- Cota D, Proulx K, Smith KA, Kozma SC, Thomas G, Woods SC, Seeley RJ. 2006 Hypothalamic mTOR signaling regulates food intake. Science 312: 927-930.
- Blouet C, Schwartz GJ. 2012 Brainstem nutrient sensing in the nucleus of the solitary tract inhibits feeding. Cell Metab 16: 579-587.
- Kim EK, Miller I, Aja S, Landree LE, Pinn M, McFadden J, Kuhajda FP, Moran TH, Ronnett GV. 2004 C75, a fatty acid synthase inhibitor, reduces food intake via hypothalamic AMP-activated protein kinase. J. Biol. Chem. 279: 19970-19976.
- Ibrahim N, Bosch MA, Smart JL, Qui J, Rubinstein M, Ronnekleiv OK, Low MJ, Kely MJ. 2003 Hypothalamic proopiomelanocortin neurons are glucose responsive and express K(ATP) channels. Endocrinology 144:1331-1340.
- Parton LE, Ye CP, Coppari R, Enriori PJ, Choi B, Zhang C-Y, Xu C, Vianna CR, Balthasar N, Lee CE, Elmquist JK, Clwley MA, Lowell BB. 2007 Glucose sensing by POMC neurons regulates glucose homeostasis and is impaired in obesity. Nature 449: 228-232.
- Farr OM, Li CR, Mantzzoros CS. 2016. Central nervous system regulation of eating: insights from human brain imaging. Metabolism 65: 699-713.
- Freeman ME, Kanyicska B, Lerant A, Nagy G. 2000 Prolactin: Structure, function, and regulation of secretion. Phys Rev 80: 1523-1631.
- Dubois-Dauphin M, Armstrong W, Tribollet E, Drefuss JJ. 1985 Somatosensory systems and the milk-ejection reflex in the rat. I. Lesions of the mesencephalic lateral tegmentum disrupt the reflex and damage mesencephalic somatosensory connections. Neuroscience 15: 1111-1129.
- Dubois-Dauphin M, Armstrong W, Tribollet E, Drefuss JJ. 1985 Somatosensory systems and dthe milk-ejection reflex in the rat. II. The effects of lesions in the ventroposterior thalamic complex, dorsal columns and lateral cervical nucleus-dorsolateral funiculus. Neuroscience 15: 1131-1140.
- Crowley WR. 2015 Neuroendocrine regulation of lactationand milk production. Compr Physiol 5:255-291.
- Li C, Chen P, Smith MS. 1999 Neural populations in the rat forebrain and brainstem activated by the suckling stimulus as demonstrated by cFos expression. Neuroscience 94: 117-129.
- Grattan DR. 2002 Behavioral significance of prolactin signaling in the central nervous sysem during pregnancy and lactation. Reproduction 123: 497-506.
- Ben-Jonathan N, Hnasko R. 2001 Dopamine as a prolactin (PRL) inhibitor. Endocr Rev 22: 724-763.
- Romano Y, Yip SH, Hodson DJ, Guillou A, Parnaudeau S, Kirk S, Tronche F, Bonnefort X, Le Tissier P, Bunn SJ, Grattan DR, Mollard P, Martin AO. Plasticity of dopamine neurons during lactation results in dissociation of electrical activity and release. J Neurosci 33: 4424-4433.
- Walsh RJ, Slaby FJ, Posner BI. 1987 A receptor-mediated mechanism for the transport of prolactin from blood to cerebrospinal fluid. Endocrinology 120: 1846-1850.
- Pi XJ, Grattan DR. 1999 Increased expression of both short and long forms of prolactin receptor RNA in hypothalamic nuclei of lactating rats. J Neuroend 11: 693-705.
- Bridges RS, Rigero BA, Byrnes EM, Yang L, Walker AM. 2001 Central infusions of the recombinant human prolactin receptor antagonist, S179D-PRL, delay the onset of maternal behavior in steroid-primed, nulliparous female rats. Endocrinology 142: 730-739.
- Yamada S., Uenoyama Y, Kinoshita M, Iwata K, Takase K, Matsui H, Adachi S, Inoue K, Maeda K-I, Tsukamura H. 2007 Inhibition of metastin (kisspeptin-54)-GPR54 signaling in the arcuate nucleus-median eminence region during lactation in rats, Endocrinology 148: 2226-2232.
- Smith MS, True C, Grove KL. 2010 The neuroendocrine basis of lactation-induced suppressionof GnRH: role of kisspeptin and leptin. Brain Res 1364: 139-152.
- Li C, Chen P, Smith MS. 1998 The acute suckling stimulus induces expression of neuropeptide Y (NPY) in cells in the dorsomedial hypothalamus and increases NPY expression in the arcuate nucleus. Endocrinology 139: 1645-1652.
- Smith MS. 1993 Lactation alters neuropeptide-Y and proopiomelanocortin gene expression in the arcuate nucleus of the rat. Endocrinology 133: 1258-1265.
- Smith MS, Grove KL. 2002 Integration of the regulation of reproductive function and energy balance: lactation as a model. Front Neuroend 23: 225-256.
- Chen P, Li C, Haskell-Luevano C, Cone R, Smith MS. 1999 Altered expression of agouti-related protein (AGRP) and its colocalization with neuropeptide Y (NPY) in the arcuate nucleus of the hypothalamus during lactation. Endocrinology 140: 2645-2650.
- Li C, Chen PL, Smith MS. 1999 Identification of neuronal input to the arcuate nucleus (ARH) activated during lactation: implications in the activation of neuropeptide Y neurons. Brain Res 824: 267-276.
- Li C, Chen P, Smith MS. 1999 Morphological evidence for direct interaction between arcuate nucleus neuropeptide Y (NPY) neurons and gonadotropin releasing hormone (GnRH) neurons and the possible involvement of Npy Y1 receptors. Endocrinology 140: 5382-5390
- Campbell RE, French-Mullen JMH, Cowley MA, Smith MS, Grove KL. 2001 Hypothalamic circuitry of neuropeptide Y regulation of neuroendocrine function and food intake via the Y5 receptor sybtype. Neuroendocrinology 74: 106-119.
- Theodosis DT, Poulain DA, Olier SHR. 2008. Activity-dependent structural and functional plasticity of astrocyte-neuron interactions. Physiol Rev 88: 983-1008.
- Nakamura K. 2011 Central circuitries for body temperature regulation and fever. Am J Physiol Regul Integr Comp Physiol 301: R1207-1228.
- Morrison SF. May 2016 Central control of body temperature. F1000Research, Published online
- Siemens J, Kamm GB. 2018. Cellular populations and thermosensing mechanisms of the hypothalamic thermoregulatory center. Pflugers Arch 470:809-822.
- Boulant JA. 2000 Role of the preoptic-anterior hypothalamus in thermoregulation and fever, Clin Inf Dis 31: S157-167.
- Chen X-M, Hosono T, Yoda T, Fukuda Y, Kanosue K. 1998 Efferent projection from the preoptic area for the control of nonshivering thermogenesis in rats. J Physiol 512: 883-892.
- Hefco E, Krulich L, Illner P, Larsen PR. 1975 Effect of acute exposure to cold on the activity of the hypothalamic-pituitary-thyroid system, Endocrinology 97: 1185-1195.
- Cypess AM, Lehman S, Williams G, Tal I, Rodman D, Goldfine AB, Kuo FC, Palmer EL, Tseng YH, Doria A, Kolodny GM, Kahn CR. 2009 Identification and importance of brown adipose tissue in adult humans. N Engl J Med 360: 1509-1517.
- Nedergaard J, Bengtsson T, Cannon B. 2007 Unexpected evidence for active brown adipose tissue in adult humans. Am J Physiol Endocrinol Metab 293: E444-452.
- Cypess AM, Kahn CR. 2010 The role and importance of brown adipose tissue in energy homeostasis. Curr Opin Pediatr 22: 478-484.
- Tam CS, Lecoultre V, Ravussin E. 2012 Brown adipose tissue: mechanisms and potential therapeutic targets. Circulation 125: 2782-2791.
- Kanosue K, Ahang Y-H, Yanase-Fujiwara M, Hosono T. 1994 Hypothalamic network for thermoregulatory shivering, Am J Physiol 267: R275-R282.
- Nagashima K, Nakai S, Tanaka M, Kanosue K. 2000 Neuronal circuitries involved in thermoregulation, Auton Neurosci 85: 18-15.
- Bamshad M, Song CK, Bartness TJ. 1999 CNS origins of the sympathetic nervous system outflow to brown adipose tissue. Am. J. Physiol. 276: R1568-R1578.
- Yoshida K, Maruyama M, Hosono T, Nagashima K, Fukuda Y, Gerstberger R, Kanosue K. 2002 Fos expression induced by warming the preoptic area in rats, Brain Res 933: 109-117.
- Zhang Y-H, Hosono T, Yanase-Fujiwara M, Chen X-M, Kanosue K. 1997 Effect of midbrain stimulation on thermoregulatory vasomotor responses in rats, J Physiol 503: 177-186.
513 Tanaka M, Nagashima K, McAllen RM, Kanosue K. 2002 Role of the medullary raphe in thermoregulatory vasomotor control in rats, J Physiol 540: 657-664.
- Rathner JA, Owens NC, McAllen RM. 2001 Cold-activated raphe-spinal neurons inrats, J Physiol 535: 841-854.
- Nakamura K. 2011 Central circuitries for body temperature regulation and fever. Am J Physiol Regul Integr Comp Physiol 301:R1207-1228.
- Caterina MJ. 2007 Transient receptor potential ion channels as participants in thermosensation and thermoregulation. Am. J. Physiol. Regulatory Integrative Comp. Physiol. 292: 64-76.
- Jancso-Gabor A, Szolcsanyi J, Jancso N. 1970 Stimulation and desensitization of the hypothalamic heat-sensitive sructures by capsaicin in rats. J. Physiol 208: 449-459.
- Sasamura T., Sasaki M., Tohda C., Kuraishi Y. 1998 Existence of capsaicin-sensitive glutamatergic tgerminals in rat hypothalamus. Neuroreport 9: 2045-2048.
- Szelenyi Z, Hummel Z, Szolcsanyi J, Davis JB. 2004 Daily body temperature rhythm and heat tolerance in TRPV1 knockout and capsaicin-pretreated mice. Eur. J. Neurosci 19: 1421-1424.
- Song K, Wang H, Kamm GB et al. 2016. The TRPM2 channel is a hypothalamic heat sensor that limits fever and can drive hypothermia Science 353: 1393-1398.
- Lu J, Zhang Y-H, Chou TC, Gaus SE, Elmquist JK, Shiromani P, Saper CB. 2001 Contrasting effects of ibotenate lesions of the paraventricular nucleus and subparaventricular zone on sleep-wake cycle and temperature regulation, J Neurosci 21: 4864-4874.
- Watts AG. The efferent projections of the suprachiasmatic nucleus: anatomical insights into the control of circadian rhythms. In: Suprachiasmatic nucleus: the mind’s clock, Klein DC, Moore RY, Reppert SM, Eds, New York, Oxford UP, pp 77-106, 1991.
- Kolka M, Stephenson L. 1989 Control of sweating during the human menstrual cycle, Eur J Appl Physiol 58: 890-895.
- Stephenson LA, Kolka MA. 1999 Esophageal temperature threshold for sweating decreases before ovulation in premenopausal women, J Appl Physiol 86: 22-28.
- Silva NL, Boulant JA. 1986 Effects of testosterone, estradiol, and temperature on neurons in preoptic tissue slices, Am J Physiol 250: R625-R632.
- Nakayama T, Suzuki M, Ishizuka N. 1975 Action of progesterone on thermosensitive neurons, Nature 258: 80.
- McEwen BS, 1981 Neural gonadal steroid actions, Science 211: 1303-1311.
- Freedman RR. 2001 Physiology of hot flashes, Am J Human Biol 13: 453-464.
- Freedman RR. Woodward S. 1995 Altered shivering threshold in postmenopausal women with hot flashes, Menopause 2: 163-168.
- Freedman RR, Dinsay R. 2000 Clonidine raises the sweating threshold in symptomatic but not in asymptomatic postmenopausal women. Fertil Steril. 74:20-23.
- Dacks PA, Krajewski SJ, Rance NE. 2011. Activation of neurokinin 3 receptors in the median preoptic nucleus decreases core temperature in the rat. Endocrinology 152:4894-4905.
- Jayasena CN, Comminos AN, De Silva A, et al, 2014 Effects of neurokinin B administration on reproductive hormone secretion in healthy men and women. J Clin Endocrinol Metab 99: E19-E27.
- Prague JK, Roverts RE, Comminos AN, et al. 2018 Neurokinin 3 receptor antagonism rapidly improves vasomotor symptoms with sustained duration of action. Menopause 25:862-869.
- Skorupskaite K, George JT, Velldhuis JD et al. 2018 Neurokinin 3 receptor antagonism reveals roles for neurokinin B in the regulation of gonadotropin secretion and hot flashes in postmenopausal women. Neuroendocrinology 106: 148-157.
- van det Heide A, Werth E, Donjacour CE et al. 2016 Core body and skin temperature in type 1 narcolepsy in daily life; effects of sodium oxybate and prediction of sleep attacks. Sleep 2016 39: 1941-1949.
- Kluger MJ. The adaptive value of fever. In: Mackowiak PA, Ed, Fever: basic mechanisms and management, New York, Raven Press, pp 105-124, 1991.
- Duff GW. 1986 Is fever beneficial to the host: a clinical perspective, Yale J Biol Med 59: 125-130.
- Scammell TE, Saper CB. 1997 Mechanisms of CNS response to systemic immune challenge: the febrile response, Trends Neurosci 20: 565-570.
- Saper CB, NeurologicalTE, Jacobson CD, Saper CB. 1996 Distribution of fos-like immunoreactivity in the rat brain following intravenous lipopolysaccharide administration, J Comp Neurol 371: 85-103.
- Lazarus M, Yoshida K, Coppari R, Bass CE, Mochizuki T, Lowell BB, Saper CB. 2007 EP3 prostaglandin receptors in the median preoptic nucleus are critical for fever responses. Nat. Neurosci. 10: 1131-1133.
- Nakamura Y, Nakamura K, Matsumura K, Kobayashi S, Keneko T, Morrison SF. 2005 Direct pyrgenic input fromprostaglandin EP3 receptor-expressing preoptic neurons to the dorsomedial hypothalamus. Eur. J. Neurosci. 22: 3137-3146.
- Morrison SF, Nakamura K, Madden CJ. 2008 Central control of thermogenesis in mammals. Exp. Physiol. 93: 773-797.
- Blatteis CM, Sehic E, Li S. 1998 Afferent pathways of pyrogen signaling, Ann NY Acad Sci 856:95-107.
- Ek M, Kurosawa M, Lundeberg T, Ericsson A. 1998 Activation of vagal afferents after intravenous injection of interleukin-1b: role of endogenous prostaglandins, J Neurosci 18: 9471-9479.
- Huang Q-H, Hruby VJ, Tatro JB. 1998 Systemic -MSH suppresses LPS fever via central melanocortin receptors independently of its suppression of corticosterone and IL-6 release, Am J Physiol 275: R524-R530.
- Huang Q-H, Entwistle ML, Alvaro JD, Duman RS, Hruby VJ, Tatro JB. 1997 Antipyretic role of endogenous melanocortins mediated by central melanocortin receptors during endotoxin-induced fever, J Neurosci 17: 3343-3351.
- Jacobowitz DM, O’Donohue TL. 1978 -Melanocyte stimulating hormone: immunohistochemical identification and mapping in neurons of rat brain, Proc Natl Acad Sci 75: 6300-6304.
- Moore R. Y. 1999 Circadian timing, in Zigmond M.J., Bloom F.E., Landis S. C., Robetos J. L., Squire L. R. (eds), Fundamental Neuroscience, Academic Press, pp. 1189-1206.
- Kriegsfeld LJ, LeSaute J, Hamada T, Pitts SM, Silver R. 2002 Circadian rhythms in the endocrine system, in Pfaff D. W, Arnold A.P., Etgen A.M., Fahrback S.E., Rubin R.T. (eds), Hormones, Brain and Behavior, vol 2, Academic Press, pp.33-91.
- Welch DK, Lagothetis DE, Meister M, Reppert SM. 1995 Individual neurons dissociated from rat suprachiasmatic nucleus express independently phased circadian firing patterns. Neuron 14: 697-706.
- Shirakawa T, Honma S, Honma K. 2001 Multiple oscillators in the suprachiasmatic nucleus. Chronobiol Int 18: 371-387.
- Moore R.Y. 1983 Organization and function of a central nervous systems circadian oscillator: the suprachiasmatic hypothalamic nucleus. Fed. Proc 43: 2783-2789.
- Ibuka M, Inoue S-L, Kawamura H. 1977 Analysis of sleep-wakefulness rhythms in male rats after suprachiasmatic lesions and ocular enucleation. Brain Res. 122: 33-47.
- Ralph MR, Foster RG, Davis FC, Menaker M. 1990 Transplanted suprachiasmatic nucleus determines circadian rhythms. Science 247: 975-978.
- Mehta N, Cheng HY. 2012 Micro-Managing the Circadian Clock: The Role of microRNAs in Biological Timekeeping. J Mol Biol. 2012 Nov 6. doi:pii: S0022-2836(12)00855-8. 10.1016/j.jmb.2012.10.022. [Epub ahead of print]
- van Ooijen G, Millar AJ. 2012. Non-transcriptional oscillators in circadian timekeeping Trends Biochem Sci. 37:484-492.
- Mrosovsky N.1996 Locomotor activity and non-photic influences on circadian clocks. Biol Rev Camb Philos Soc 71: 343-372.
- Zee PC, Manthena P.2007 The brain’s master circadian clock: implications and opportunities for therapy of sleep disorders. Sleep Med Rev 11: 59-70.
- Tonsfeldt KJ., Chappell PE.2012Clocks on top: the role of the circadian clock in the hypothalamic and pituitary regulation of endocrine physiology. Mol Cell Endocrinol 349: 3-12.
- Cheng, M.Y., Bullock, C.M., Li, C., Lee, A.G., Bermak, J.C., Belluzzi, J., Weaver, D.R., Leslie, F.M., Zhou, Q.Y. 2002. Prokineticin 2 transmits the behavioural circadian rhythm of the suprachiasmatic nucleus. Nature 417, 405–410.
- Hamada T, LeSauter J, Venuti JM, Silaver R. 2001 Espression of Period genes: rhythmic and nonrhythmic compartments of the suprachiasmatic nucleus pacemaker. J Neurosci 21: 7742-7750.
- Ingram CD, Ciobanu R, Coculescu IL, Tanasescu R, Coculescu M, Mihai R. 1998 Vasopressin neurotransmission and the control of circadian rhythms in the suprachiasmatic nucleus. Prog Brain Res 119: 351-364.
- Jin X, Shearman LP, Weaver DR, Zylka MJ, deVries GJ, Reppert SM. 1999 A molecular mechanism regulating rhythmic output from the suprachiasmatic circadian clock. Cell 96: 57-68.
- Arima H, House SB, Gainer H, Aguilera G. 2002 Neuronal activity is required for the circadian rhythm of vasopressin gene transcription in the suprachiasmatic nucleus in vitro. Endocrinology 143: 4165-4171.
- Romijin HJ, Sluiter AA, Pool CW, Wortel J, Buijs RM. 1997 Evidence from confocal fluorescence microscopy for a dense, reciprocal innervation between AVP-, somatostatin-, VIP/peptide histidine isoleucine-, GRP, and peptide histidine isoleucine/GRP-immunoreactiave neurons in the rat suprachiasmatic nucleus. Eu J Neurosci 9: 2613-2623.
- Gillette MU, Tischkau SA. 1999 Suprachiasmatic nucleus: The brain’s circadian clock. Recent Prog Brain Res 54: 33-59.
- Moore RY.1996 Neural control of the pineal gland. Behav Brain Res 73: 125-130.
- Moore RY. 1996 Entrainment pathways and the functional organization of the circadian system.Prog. Brain Res. 111: 103-119..
- Arendt J.2000 Melatonin, circadian rhythms, and sleep. N Engl J Med. 343: 1114-1116.
- Karsch FJ, Bittman EL, Robinson JE, Yellon SM, Wayne NL, Olster DH, Kaynard AH. 1986 Melatonin and photorefractoriness: loss of response to the melatonin signal leads to seasonal reproductive transitions in the ewe. Biol Reprod. 34: 265-274.
- Azzali G, Arcari ML, Cacchini A, Toni R.2003 Fine structure and photoperiodical seasonal changes in Pars tuberalis of hibernating bats. It J Anat Embriol 108(1):49-64.
- Drew KL, Buck CL, Barnes BM, Christian SL, Rasley BT, Harris MB. 2007 Central nervous system regulation of mammalian hibernation:implications for metabolic suppression and ischemia tolerance. J Neurochem 102: 1713-1726.
- Kalsbeek A., Buijs RM.2002 Output pathways of the mammalian suprachiasmatic nucleus: coding circadian time by transmitter selection and specific targeting. Cell Tissue Res 309:109–118.
- Feillet CA.2010 Food for thoughts: feeding time and hormonal secretion. Journal of Neuroendocrinology 22, 620–628.
- Girotti, M., Weinberg, M.S., Spencer, R.L. 2009. Diurnal expression of functional and clock-related genes throughout the rat HPA axis: system-wide shifts in response to a restricted feeding schedule. Am J Physiol Endocrinol Metab 296, 888–897.
- Fuller MP, Gooley JJ, Saper CB. 2006 Neurobiology of the sleep-wake cycle:sleep architecture, circadian regulation and regulatory feedback. J Biol Rythms 21: 482-493.
- Saper CB, Chou TC, Scammell TE. 2001 The sleep switch:hypothalamic control of sleep and wakefulness. Trend Neurosci 24: 727-731.
- Sherin JE, Shiromani PJ, McCraly RW, Saper CB. 1992 Activation of ventrolateral preoptic neurons during sleep.Science 271, 216-219, 1996.
- Lugaresi E.1992 The thalamus and insomnia. Neurology 42: 28-33.
- Steriade M.1994 The thalamus and sleep disturbances. In: Guilleminault C, Lugaresi E, Montagna P, Gambetti P, eds, Fatal Familial Insomnia: Inherited Prion Diseases, Sleep and the Thalamus. New York, Raven Press, pp. 177-189.
- Moruzzi G, Magoun HW.1949 Brain stem reticular formation and activation of EEG. Electroecaphalogry Clin Neurol 1: 455-473.
- Toni R, Malaguti A, Benfenati F, Martini L. 2004 The human hypothalamus: a morphofunctional perspective.J Endocrinol Invest 27 (supp to n.6): 73-94.
- Sherin JE, Elmquist JK, Torrealba F, Saper CB. 1998 Innervation of histaminergic tuberomammillary neurons by GABAergic and galaninergic neuros in the ventrolateral preoptic nucleus of the rat.J Neurosci 18: 4705-4721.
- Ericson H,Blomqvist A, Köhler C. 1991 Origin of neuronal inpus to the region of 6he tuberomammliary nucleus of the rat brain. J Comp Neurol 311: 45-64.
- Yoshimoto Y, Sakai K, Luppi PH, Fort P, Salvert D, Jouvet M. 1989 Forebrain afferents to the cat posterior hypothalamus:a double labelling study. Brain Res Bull 23: 83-104.
- Gervasoni D, Peyron C, Rampon C, Barbagli B, Chouvet G, Urbain N, Fort P, Luppi PH. 2000 Role and origin of the GABAergic innervation of dorsal raphé serotoninergic neurons. J Neurosci 20: 4217-4225.
- Steininger TL, Gong H, McGinty D, Szymusiak R. 2001 Subregional organization of preoptic area/anterior hypothalamic projections to arousal-related monaminergic cell groups.J Comp Neurol 429: 638-653.
- Parent A, Steriade M. 1984 Midbrain tegmental projections of nucleus reticularis thalami of cat and monkey:a retrograde transport and antidromic invasion study. J Comp Neurol 229: 548-558.
- Saper CB. 2000 Hypothalamic connections with the cerebral cortex.Prog Brain Res 126: 40-38.
- Fuller PM, Sherman D, Pedersen NP, Saper CB, Lu J. 2011 Reassessment of the structural basis of the ascending arousal system. J Comp Neurol. 519: 933–956.
- Saper CB, Scammell TE, Lu J. 2005 Hypothalamic regulation of sleep and circadian rhythms.Nature 437: 1257-1263.
- Buzsaki G, Bickford RG, Ponomareff G, Thal LJ, Mandel R, Gage FH. 1988 Nucleus basalis and thalamic control of neocortical activity in the freely moving rat.J Neurosci 8: 4007-4026.
- Kampfl A, Franz G, Aichner F, Pfausler B, Haring HP, Felber S, Luz G, Schocke M, Schmutzhard E.1998 The persistent vegetative state after closed head injury: clinical and magnetic resonance imaging findings in 42 patients. J Neurosurg. 88:809-816.
- Manford M, Andermann F. 1988 Complex visual hallucinations. Clinical and neurobiological insights. Brain.121:1819–1840.
- Aschkenasi CJ, Lee CE, Chemelli RM, Saper CB, Yanagisawa M, Elmquist JK. 2001 Differential expression of orexin receptors 1 and 2 in the rat brain.J Comp Neurol 435: 6-25.
- Trivedi P, Yu H, MacNeil DJ, Van der Ploeg LH, Guan XM. 1998 Distribution of orexin receptor mRNA in the rat brain.FEBS Lett 438: 71-75.
- Hagan JJ, Leslie RA, Patel S, Evans ML, Wattam TA, Holmes S, Benham CD, Taylor SG, Routledge C, Hemmati P, Munton RP, Ashmeade TE, Shah AS, Hatcher JP, Hatcher PD, Jones DN, Smith MI, Piper DC, Hunter AJ, Porter RA, Upton N. 1999 Orexin A activates locus coeruleus cell firin and increases arousal in the rat.Proc. Natl Acad Sci USA 96: 10911-10916.
- Brown RE, Sergeeva O, Eriksson KS, Haas HL. 2001 Orexin A excites serotoninergic neurons in the dorsal raphé nucleus of the rat.Neuroparmacology 40; 457-459.
- Methippara MM, Alam MN, Szymusiak R, McGinty D. 2000 Effects of lateral preoptic area application of orexin-A on sleep-wakefulness.NeuroReport 11: 3423-3426.
- Chemelli RM, Willie JT, Sinton CM, Elmquist JK, Scammell T, Lee C, Richardson JA, Williams SC, Xiong Y, Kisanuki Y, Fitch TE, Nakazato M, Hammer RE, Saper CB, Yanagisawa M. 1999 Narcolepsy in orexin knockout mice: molecular genetics of sleep regulation.Cell 98: 437-451.
- Lin L, Faraco J, Li R, Kadotani H, Rogers W, Lin X, Qiu X, de Jong PJ, Nishino S, Mignot E. 1999 The sleep disorder canine narcolepsy is caused by a mutation of the hypocretin (orexin) receptor 2.Cell 98: 365-376.
- Nishino S, Ripley B, Overeem S, Lammers GJ, Mignot E. 2000 Hypocretin (orexin) deficiency in human narcolepsy.Lancet 355: 39-40.
- Kocsis B, Kaminski M. 2006 Dynamic changes in the direction of theta rhythmic drive between supramammillary nucleus and the septohippocampal system.Hippocampus 16: 531-540.
- Lu J, Sherman D, Devor M, Saper CB. 2006 A putative flip-flop switch for control of REM sleep.Nature 441: 589-594
- Lugaresi E, Montagna P. 1994 Thalamus, sleep and circadian funtions.In: Guilleminault C, Lugaresi E, Montagna P, Gambetti P, eds, Fatal Familial Insomnia: Inherited Prion Diseases, Sleep and the Thalamus. New York, Raven Press, pp 215-219.
- Marini G, Imeri L, Mancia M. 1988 Changes in sleep-waking cycle induced by lesions of medial dorsalis thalamic nuclei in the cat.Neurosci Lett 85: 223-227.
- Lugaresi E, Medori R, Montagna P, Baruzzi A, Cortelli P, Lugaresi A, Tinuper P, Zucconi M, Gambetti P. 1986 Fatal familial insomnia and dysautonomia with selective degeneration of thalamic nuclei.New Engl J Med 315: 997-1003.
- Lu J, Shang YH, Chou T, Gauss SE, Elmquist JK, Shiromani P, Saper CB.2001 Contrasting effects of ibotenate lesions of the paraventricular nucleus and subparaventricular zone on sleep-wake cycle and temperature regulation. J Neurosci 21: 4864-487.
- Gooley JJ, Schomer A, Saper CB. 2006 The dorsomedial hypothalamic nucleus is critical for the expression of food-entrainable circadian rhythms.Nat Neurosci 9: 398-407.
- Chou TC, Scammell TE, Gooley JJ, Gaus SE, Saper CB, Lu J. 2003 Critical role of dorsomedial hypothalamic nucleus in a wide range of behaviorl circadian rhythms.J Neurosci 23: 10691-10702.
- Saper CB, Lu J, Chou TC, Gooley J. 2005 The hypothalamic integrator of circadian rhythms.Trends Neurosci 28: 152-157.
- Harrington ME. 1997 The ventral lateral geniculate nucleus and the intergeniculate leaflet: interrelated structures in the visual and circadian systems.Neurosci Biobehav Rev 21; 705-727.
- Donlea JM, Thimgan MS, Suzuki Y, Gottschalk L, Shaw PJ. 2011Inducing sleep by remote control facilitates memory consolidation in Drosophila. Science. 332:1571-1576.
- Elbaz I, Foulkes NS,Gothilf Y, Lior Appelbaum L. 2013Circadian clocks, rhythmic synaptic plasticity and the sleep-wake cycle in zebrafish.Frontiers in Neural Circuits 7: 1-7.
- Saper CB, Fuller PM, Pedersen NP, Lu J, Scammell TE. 2010Sleep state switching. Neuron. 68:1023-1042.
- Nofzinger EA, Buysse DJ, Germain A, Price JC, Miewald JM, Kupfer DJ. 2004Functional neuroimaging evidence for hyperarousal in insomnia. Am J Psychiatry. 161:2126–2128.
- Porkka-Heiskanen T, Strecker RE, Thakkar N, Bjiorkum AA, Greene RW, McCarley RW. 1997 Adenosine:a mediator of sleep-inducing effects of prolonged wakefulness. Science 276, 1265-1268.
- Shilber U. 2007 The daily timing of gene expression and physiology in mammals.Dialogues Clin Neurosci 9: 257-272.
- Naylor E, Bergman BM, Krauski K, Zee PC, Takahashi JS, Vitaterna MH, Turek FW.2000 The circadian clock mutation alters sleep homeostasis in the mouse. J Neurosci 20: 8138-8143.
- Wisor JP, O’Hara BF, Terao A, Selby CP, Kilduff TS, Sancar A, Edgar DM, Franken P.2002 A role for cryptochromes in sleep regulation. BMC Neurosci 3: 20.
- Lapolsky A, Easton A, Dugovic C, Walisser J, Bradfield C, Turek F. 2005 Deletion of the mammalian circadian clock gene Bmal1/Mop3 alters baseline sleep architecture and the response to sleep deprivation.Sleep 2: 395-409.
622 .Sahar S, Sassone-Corsi P. 2012 Regulation of metabolism: the circadian clock dictates the time. Trends Endocrinol Metab. 3: 1–8.